Benefits of physical exercise on Alzheimer's disease: an epigenetic view
Abstract
Increasing lines of evidence have indicated the beneficial impacts of exercise on the neurodegeneration and cognitive decline of Alzheimer’s disease (AD). While general mechanisms underlying the positive effects, including the elevated neurotrophins level, improved neurogenesis and neuroplasticity, restored angiogenesis and autophagy, and reduced neuroinflammation, have been well documented, the epigenetic mechanisms of exercise on AD, however, are still inconclusive. Exercise can regulate the expression of those AD-related genes or proteins through various epigenetic modulations, thereafter rescuing AD pathologies and improving cognitive deficits of AD. In this review, we briefly summarized recent research advances in the beneficial impacts of exercise on cognition and AD and discussed the underlying mechanisms from an epigenetic point of view, including DNA methylation, histone modifications, and non-coding RNAs. A deep understanding of how exercise epigenetically promotes cognitive and pathological recoveries in AD is crucial for the future discovery of precise exercise procedures or exercise-like remedies to treat this disease.
Keywords
INTRODUCTION
Exercise, a subset of physical activity, has been recognized as an important approach to maintaining or improving the health of our body including the brain. Increasing lines of evidence have supported the positive impacts of exercise on emotional and cognitive performance during brain aging, whereas physical inactivity has been considered a risk factor for the incidence and progression of various psychiatric disorders[1,2] and neurodegenerative diseases[3,4]. The neuroprotective effects and general benefits yielded from physical exercise might be due to improved neurogenesis and neuroplasticity, recovered angiogenesis and autophagy, increased neurotrophin secretion, and reduced neuroinflammation[5]. Moreover, recent studies indicate that the positive impacts of exercise on brain function may be achieved through not only those above-mentioned mechanisms but also an epigenetic approach. The roles of epigenetics have gained more and more attention considering its modulating activity to alter chromatin and gene transcription so as to influence brain function. While the benefits of exercise to improve cognition and combat neurodegeneration, including Alzheimer’s disease (AD), have been comprehensively summarized and discussed in previously published reviews[6-10], very limited literature has focused on the epigenetic mechanisms underlying the effects of exercise against AD-related phenotypes and pathologies. In this review, we briefly summarized research advances in the benefits of exercise on brain health and cognition and discussed potential mechanisms underlying the benefits of exercise on AD from an epigenetic point of view.
EPIGENETIC ALTERATIONS IN ALZHEIMER’S DISEASE
Epigenetics
The term epigenetics is derived from the Greek word and mean over or above the genome. In biology, epigenetics was coined in 1942 by Conrad H. Waddington to define the process of how environmental factors regulate gene expression without changing DNA sequence. Epigenetic modifications are essential for many biological processes, especially during early life development and specialization, and may be maintained through cell divisions or inherited through generations. On the contrary, improper epigenetic modulations can result in pathological consequences and are involved in many human diseases. Epigenetic processes are thought to influence gene expression chiefly at the transcriptional and post-transcriptional levels. The common epigenetic modifications include DNA methylation, histone modifications, and non-coding RNAs (ncRNAs) regulation. DNA methylation normally associates with gene silencing when the methylation occurs in cytosine-phosphate-guanine (CpG) islands of promoter sequences. Histone modifications lead to the open euchromatin state, which facilitates gene expression, or the closed heterochromatin state, which suppresses gene transcription. In addition, ncRNAs have also been shown to play a key role in the regulation of gene expression.
DNA methylation
DNA methylation is a major epigenetic modification consisting of the covalent addition of a methyl group, transferred from S-adenosylmethionine to cytosine residue within CpG and non-CpG dinucleotide sites, leading to the formation of 5-methyl cytosine (5mC). DNA methylation is catalyzed by a family of DNA methyltransferases (DNMTs)[11], resulting in methylation of the DNA and the subsequent alterations of gene expression[12]. There are five members in the family of DNMTs, including DNMT1, DNMT2, DNMT3A, DNMT3B, and DNMT3L[13]. While DNMT1, DNMT3A and DNMT3B are canonical members of DNMTs catalyzing the addition of methylation groups to genomic DNA bases, DNMT2 and DNMT3L are non-canonical family members with relatively low catalytic DNMT activity[13]. However, DNMT3L binds to DNMT3A or DNMT3B to form heterodimers which promote the catalytic activity of DNMT3A and DNMT3B[13].
Oppositely, the level of DNA methylation can be downregulated by DNMTs inhibitors or active removal of 5mC. Ten-eleven translocation (TET) proteins catalyze the conversion of 5mC into
Histone modifications
Chromatin structure and gene accessibility to transcriptional machinery is regulated by modifications to histone tails. The N-terminal tails of histone protein in nucleosomes can be epigenetically modified through phosphorylation, ubiquitination, sumoylation, acetylation and methylation. All these epigenetic modifications modulate the chromatin structure and function to control the transcription and translation of specific genes.
Among all types of histone modifications, the histone acetylation at the ε-amino group of lysine residues in H3 and H4 tails is most consistently associated with the promotion of transcription, whereas the deacetylation of histones correlates with CpG methylation and chromatin inactivation. Compared to acetylation, the impact of histone methylation on transcription is much more complicated. The methylation in the histone N-terminal tail can either promote or repress specific gene expression, depending on the amino acid residue being modified and also on the type of modifications (monomethylated, dimethylated, or trimethylated). For example, the methylation of histone H3 at different amino acid residues leads to contradictory regulation. The methylations on lysine 4 and lysine 36 are associated with transcriptional activation, whereas those on lysine 9 and lysine 27 are associated with transcriptional repression[18].
ncRNAs regulation
ncRNAs are RNA molecules that are transcribed from genomic DNA but do not encode proteins. ncRNAs play essential roles in the epigenetics regulation of gene expression in addition to their roles at the transcriptional and post-transcriptional levels. Interestingly, ncRNAs are particularly abundant in the central nervous system and the altered ncRNAs profile has been closely correlated with brain aging and neurodegeneration[19,20]. ncRNAs can be categorized into small ncRNAs (sncRNA, < 200 nucleotides) and long ncRNAs (lncRNA, > 200 nucleotides). sncRNAs, including microRNAs (miRNAs) and piwi-interacting RNAs (piRNAs), modify chromatin structure and silence transcription by guiding Argonaute-containing complexes to complementary nascent RNA scaffolds and then mediating the recruitment of histone methyltransferases and DNMTs. In contrast, lncRNAs control chromatin structure mainly via interacting with nucleosome remodeling factors as well as chromatin-modifying enzymes.
Epigenetic modifications in cognition
In the brain, increasing lines of evidence, either from animal models or human subjects, have implied the involvement of epigenetic modifications in the biological processing of cognition, especially memory formation and consolidation.
DNA methylation and cognition
While the involvement of DNA modifications in memory storage was first proposed in 1969, early studies have further revealed changes in DNA methylation of genes in the hippocampus during learning and memory. Specifically, DNMTs expression is upregulated in the hippocampus following contextual fear conditioning and DNMT is required for the consolidation and reconsolidation of memory-associated neural plasticity[21,22]. Moreover, inhibiting DNMTs expression disrupts contextual fear memory formation, blocks memory maintenance, or improves short-term object pattern separation memory, via modifying the methylation of specific memory-related genes including the brain-derived neurotrophic factor (BDNF), Protein phosphatase 1 and Reelin[23-27].
Notably, while experimental evidence has confirmed the close relationship between DNA methylation or its catalytic enzymes with cognition, the global level of DNA methylation is failed to be correlated with cognitive performance in either healthy older adults or 4 years old children[28-29]. This conflict may highlight a possible involvement of other factors in epigenetic modulations of cognition, especially under pathological conditions such as brain aging and neurodegeneration.
Histone modifications and cognition
Besides DNA methylation, increasing lines of evidence have also revealed important roles of histone modifications such as methylation, acetylation, ubiquitination, or phosphorylation in neuronal plasticity and memory processing[30-33].
Previous studies investigated the contribution of histone methylation to memory formation. Gupta et al found that trimethylation of histone H3 at lysine 4 (H3K4me3), an active mark for transcription, and dimethylation of histone H3 at lysine 9 (H3K9), a silencing mark for transcription, was upregulated in the hippocampus of rats subjected to contextual fear conditioning. In addition, mice deficient in the H3K4-specific histone methyltransferase displayed impaired contextual fear conditioning, suggesting the involvement of histone methylation in long-term memory consolidation[34], which was further supported by other studies[35-37]. Despite the hippocampus, H3K9-mediated transcriptional repression has been found to be required for fear-related memory in other brain regions, such as the entorhinal cortex and amygdala[38,39].
Histone acetylation is also associated with memory and cognition[40,41]. H3 acetylation is rapid and reversible, being controlled by histone acetyltransferases (HATs) and deacetylases (HDACs). HATs activity deficiency leads to impaired learning and memory performance in mice[42,43]. In contrast to HATs, HDACs seem to regulate cognition in an inconsistent way. For Class I HDACs, HDAC1 normally plays a role in memory extinction, whereas HDAC2 and HDAC3 negatively regulate learning and memory[44]. As for the class II HDACs, HDAC4 deficiency was found to be correlated to improved memory in C. elegans[45], while HDAC4 deletion induced memory impairment in mice [46-47]. Moreover, the lack of HDAC5 in mice disrupts the memory process at 10 months of age but not 2 months[48].
Histone phosphorylation is another epigenetic modification regulating cognitive function. Gräff et al. found a rapid H3S10 phosphorylation of histone after the object memory test[49], which was consistent with previous reports showing an increased histone phosphorylation shortly after fear conditioning[50]. Moreover, germline knockout of mitogen-and stress-activated protein kinase 1 (MSK1), a key modulator of histone phosphorylation, impairs long-term spatial and contextual fear memory formation, leaving cued fear memory intact[51]. In addition to MSK1, IκB kinase-α also regulates histone phosphorylation[52] and appears to be involved in the reconsolidation of conditioned fear memories[53].
Moreover, although rarely investigated, recent evidence also suggests a potential contribution of histone ubiquitination in memory formation. Previous studies indicated that the contextual fear conditioning paradigm increased global and gene-specific histone H2B lysine 120 mono-ubiquitination (H2BubiK120) levels in rat hippocampus. Loss of H2BubiK120 impaired LTP and memory formation, which could not be rescued by upregulation of H3K4me3[54].
Notably, various epigenetic modifications involved in cognition usually co-exist with each other rather than work independently. For example, the acetylation of H3K14 works together with the phosphorylation of serine 10 and the trimethylation of H3K36, and H3 acetylation co-occurs with DNA methylation, working together to regulate the memory procecss[32,47,55-58]. It may be worth investigating how a cross-talk or up/down-stream regulation network functions among these co-occurring epigenetic modifications, thereafter, to deepen our current knowledge in the epigenetic regulation of cognition.
ncRNAs regulation in cognition
ncRNAs and their associated functional networks have been implicated in regulating complicated neurobiological processes including cognition and behavior[59-61]. An extensive body of evidence has demonstrated the clear correlations between miRNAs and neuronal activity. Neuronal activity regulates the expression and turnover of neural miRNAs, which in turn permits local translation of mRNAs encoding synaptic proteins at dendritic spines and postsynaptic densities necessary for synaptic function. For example, a specific group of miRNAs, including miR-132, is increased in response to neuronal activity and may ultimately promote the CREB/BDNF signaling-mediated elevation of dendritic spine formation and maturation[62]. However, in another study, miR-132 overexpression decreases MeCP2 expression and impairs cognitive performance in mice. In addition, the brain-specific miR-134 depressed CREB and BDNF expression, thereby impairing synaptic plasticity and recognition performance[63]. Moreover, miR-138-5p, another brain-enriched miRNA, can serve as an important regulator of short-term memory and inhibitory synaptic transmission in the mouse hippocampus[64]. piRNA, another subtype of sncRNA, induces endured epigenetic changes, which can be inherited across many generations and underlie mechanisms of activity-dependent, adaptive memory storage[65]. For example, aca-piR-F in Aplysia increases piwi/piRNA-dependent methylation at the CREB2 promoter and suppresses CREB2 expression, leading to enhanced long-term synaptic facilitation[66]. Another study found that selective deficiency of PIWI proteins in mice hippocampus results in enhanced contextual fear memory, further supporting the involvement of piRNAs in the regulation of memory[67].
In contrast to those above-mentioned sncRNAs, lncRNA profiles also appear to be correlated with cognition. Intriguingly, although lncRNAs can be found throughout the body, an estimated 40% expresses specifically in the brain, suggesting brain-specific roles for lncRNAs[68]. With advances in high throughput sequencing technologies and functional profiling methods, lncRNAs have been functionally and mechanistically correlated with neurobiological processes responsible for cognition. Previous studies have shown that nuclear lncRNAs may play important roles in the regulation of genes in charge of regulating synaptic plasticity and memory. For example, RNAseq analysis demonstrated an activity-dependent regulation of the lncRNA Gomafu in the medial prefrontal cortex (mPFC) after cued fear conditioning. Knockdown of Gomafu in the mPFC impaired the acquisition of fear responses in cued fear conditioning[69]. Wen et al. found that the down-regulation of lncRNA ANRIL in hippocampal pyramidal neurons ameliorated learning and memory deficits in diabetic rats via the NF-kB signaling pathway[70]. In contrast, NEAT1, a highly abundant nuclear architectural lncRNA, mediates neuronal histone methylation and age-associated memory impairment[71]. In addition to nuclear lncRNAs, cytoplasmic lncRNAs have also been implicated to be involved in synaptic plasticity changes in memory. For instance, lncRNA Durga has been demonstrated to be an important regulator for modulating dendritic morphology and kalirin expression in zebrafish[72].
All these findings provide compelling evidence to support the pivotal roles of ncRNAs in cognition. Further studies are still required to elucidate the consequences of precise spatio-temporal depletion or overexpression of candidate ncRNAs. The yielded data will not only help expand our current knowledge about the exact epigenetic modulating network in cognition but also shed insight into future therapy of diseases with impaired cognition, such as AD.
Epigenetic alterations in Alzheimer’s disease
Impressive achievements have been made in understanding the possible mechanisms for AD pathogenesis and progression. It is well-accepted that AD is a multifaceted disease mediated by interactions between genetic and environmental factors. Currently, most of our knowledge about AD is based on fundamental research works using transgenic animal models carrying artificial genetic backgrounds, which only partially reflect the genetic aspect of this complicated disease. This limitation facilitates our investigations to detect other possible mechanisms to fully understand AD.
Although genetic factors appear to play pivotal roles in the etiology and pathogenesis of early-onset AD[73], epigenetic modifications have gained more attention, especially for late-onset AD[74-77]. More than 20 epigenetic mechanisms have been identified to be correlated to AD, most of which involve DNA methylation, histone modifications [partially summarized in Table 1], or modifications of mRNA-related processes, including ncRNA and miRNA. Various aversive environmental AD risk factors can induce epigenetic modifications of key genes and pathways related to AD and contribute to AD onset. Several factors that have been associated with AD, such as diabetes mellitus, high blood pressure, obesity, diet, excessive sedentary lifestyle, smoking, and even a low educational level, are capable of inducing epigenetic changes. In contrast, a good lifestyle including proper physical exercise, sufficient sleep, vigorous emotional status, proper diet or nutrient supplement and social interactions is currently considered to prevent the pathogenesis of disease, delay the progression, or reduce the severity of AD. Among these daily life factors, physical exercise has been reported as one of the most accessible and feasible approaches.
Epigenetic alterations in the brain of Alzheimer’s disease
Epigenetic alterations | Target genes (proteins) | Animal models or human subjects | References |
DNA methylation | HOXA3 and ANK1 | Postmortem human brain tissue in AD patients | [78] |
IL6 and SIAH1 | Postmortem human brain tissue and blood samples in AD patients. | [79] | |
ADAM10 | Whole blood DNA from AD patients | [80] | |
APP | Human brain tissues of AD patients | [81,82] | |
BACE1 | Prefrontal cortex neurons of AD patients | [83] | |
ApoE4 and PIN1 | Human brain tissues of AD patients | [84,85] | |
GSK3β and PP2A | TgCRND8 mice | [86] | |
ANK1 and WNT5B | Entorhinal cortex of the brain from AD patients | [87] | |
RIN3, CTSG, SPEG and UBE2L3 | Peripheral blood samples from patients with MCI | [88] | |
BRCA1 and AURKC | Postmortem brains of AD patients | [89] | |
ANK1 | Postmortem brains of AD patients | [90,91] | |
HOXA3, GSTP1, CXXC1-3 and BIN1 | Post-mortem PFC of AD patients (LOAD) | [92] | |
TNF-α | Cortex samples from 4 healthy subjects and 4 AD | [93] | |
HOXA | brain tissues from AD patients | [94] | |
PSD95 | APP/PS1 mice | [95] | |
PM20D1 | APP/PS1 mice | [96] | |
Histone acetylation | BACE1, | 3xTg-AD mice brain and PBMC from AD patients | [97] |
BACE1 and PS1 | APP overexpressed N2a cells | [98] | |
PS1 and PS2 | Swiss albino mice | [99] | |
APP | entorhinal cortex samples from AD cases | [100] | |
DNA methylation and histone acetylation | BIN1 | Human cortical brain tissue | [101] |
Txnip | 3xTg-AD mice | [102] |
DNA methylation in Alzheimer’s disease
Although the global DNA methylation pattern is failed to be correlated with cognitive performance in healthy human subjects, an altered DNA methylation has been observed in AD brain[103]. Moreover, DNA methylation alterations have also been identified in candidate genes that were closely correlated with AD pathophysiology. For example, in AD, the expression of PSEN1, BACE1 and APP genes, all involved in Aβ production and AD pathology, are promoted by hypomethylation of these gene promoters[75,104]. In addition, hyper-methylation has been associated with a higher presence of the APOE ε4 allele, the strongest genetic risk factor for late-onset AD[105]. As for the neurofibrillary tangles formed by aggregates of hyperphosphorylated tau protein, another pathological hallmark of AD, methylations-mediated epigenetic modulations have also been observed. Hypomethylation of glycogen synthase kinase 3β (GSK3β) promoter results in the overexpression of GSK3β, leading to tau hyperphosphorylation. In contrast, the hypomethylation of protein phosphatase 2A (PP2A) results in reduced activity of PP2A, thus cannot properly dephosphorylate tau, leading to tau hyperphosphorylation[86].
Besides those above-mentioned well-accepted AD risk genes, De Jager et al. also found a close correlation between AD pathology and DNA methylation, especially in the regions of ATP-binding cassette A7 and bridging integrator 1 genes, both of which harbor AD susceptibility alleles[106]. Interestingly, the methylation changes appeared in the early stage of the disease, as evidenced by the fact that these changes appeared in patients with characteristic amyloid pathology, even if they had not yet developed cognitive impairment. In another study, researchers performed a cross-tissue analysis of methylomic profile using AD brain and blood samples from different regions in four independent cohorts. They identified a differentially methylated region in the ankyrin 1 gene that was associated with neuropathology in the entorhinal cortex, a primary site of AD manifestation[107].
The DNA methylation status in the hippocampus is further determined, considering its pivotal role in cognition. Chouliaras et al. found that, compared with controls, AD patients showed a reduced 5mC level in hippocampus, which was negatively correlated to both amyloid plaque and tangle in the hippocampus[108]. More specifically, the decreased 5mC was found in both neuronal and glial cells in the CA1 region of hippocampus, whereas only glial cells in the CA3. Moreover, global DNA hypomethylation has been reported to be accompanied by decreased DNMT1 and DNMT3A expression in the hippocampus of postmortem AD samples[109].
DNA methylation alterations have also been observed in the frontal cortex of AD brain, but the findings are somehow inconsistent. For instance, DNA hypomethylation of CpG sites in exon promoter region was observed in the superior frontal gyrus of prefrontal cortex in AD[110]. In contrast, DNA methylation levels were increased in the medial frontal gyrus and were positively correlated with AD pathology[111]. The complexity of the DNA methylation changes in the AD brain was investigated by genome-wide methylation analysis[112]. The results revealed bidirectional DNA methylation alterations in a gene-specific manner; that is, hypermethylated genes were largely related to the regulation of transcription and gene expression, while genes with hypomethylation levels of CpG sites in promoter region were largely related to protein metabolism and membrane transport[112].
Considering the multifaceted nature of AD pathogenesis under complex interplays between environmental and genetic factors, this disease is regulated by a complicated and precisely controlled spatiotemporal gene expression network, which is in turn affected by epigenetic mechanisms including DNA methylation. Although the migration of AD pathologies is temporally and regionally specific according to Brrak staging, it is worth investigating the spatio-temporal profiles of these epigenetic changes, in order to clarify the exact epigenetic modulating network in AD. To reach this goal, multi-omics studies involving spatial omics are a promising direction in the future[113,114].
Histone modifications in Alzheimer’s disease
Histone modifications are also important regulatory pathways in the development and progression of AD. Previous studies have revealed a close relationship between AD and histone acetylation. For example, acetylation of H4 was decreased in APP/PS1 mouse hippocampus. Administration of the HDAC inhibitors rescued the deficit in H4 acetylation and improved cognitive function in AD animal models[115,116]. In addition, HDAC6 inhibitor also blocked the Aβ-induced impairment of mitochondria transport in hippocampal neurons[117], inhibited HDAC6-dependent tubulin deacetylation in the mouse hippocampus, restored impaired axonal transport and novel object recognition in the P301S tau transgenic mouse, and decreased RIPA-insoluble tau accumulation[118]. However, similar to DNA methylation, bidirectional changes in histone acetylation in AD can also be observed. For example, while studies found increased activity of HDAC2 in the brains of patients with AD[119], another study observed downregulated histone marks in quantitative states of H3K18/K23 acetylation[120].
Histone methylation is another best-studied histone modification. In contrast to histone acetylation, although histone methylation has been implicated with cognition[32], the roles of histone methylation in AD are still rarely investigated. One clinical study identified methylation of H2B K108 and H4R55 in the frontal cortex of AD patients[121]. In addition, a postmortem study of AD brain reported an elevated level of H3K9me2 protein in the occipital cortex compared to non-demented and age-matched controls[122]. Much more recently, Persico et al. found a lower H3K4me3 and higher H3K27me3 level in the entorhinal cortex of patients with AD, compared with age-matched control subjects[123]. Consistent with these clinical findings, histone modifications have also been identified in AD animal models. For instance, significant elevation of H3K9me2 and Emt1 (G9a) and Emt2 (GLP) in the prefrontal cortex and hippocampus from the aged AD mouse model are accompanied by reduced glutamate receptor transcription and AD-like cognitive deficits[124]. Moreover, a loss of nuclear H3K4me3 in the hippocampus was found in the 3xTg AD mouse model, while an increase in H3K4me3 and Kmt2a was found in P301S transgenic Tau mice (line PS19)[125]. These epigenetic findings from either clinical or animal studies provide robust experimental evidence to support the involvement of histone methylation in AD. Restoring the homeostasis of histone methylation may be a potential therapeutic strategy to treat AD.
ncRNAs alterations in Alzheimer’s disease
ncRNAs are essential for the proper maintenance of cognitive function and have been represented as important epigenetic mechanisms associated with AD pathogenesis[126-128]. Their expression occurs in a variety of genomic regions important for APP processing, Aβ production, tau pathology, and neurodegeneration. Their regulatory functions are thought to depend on brain development and cell differentiation, as well as on various environmental factors related to AD[129-131].
miRNAs are endogenous sncRNAs regulating gene expression by inhibiting the transcription or inducing degradation of mRNA. Impairment of miRNA-epigenetic regulatory pathway can disrupt chromatin function and consequently lead to neurodegeneration[132-134]. For example, miR-221, miR-144 and miR-374 levels were decreased in the brains of AD patients compared to healthy controls, and the circulating miR-137, miR-181c, miR-9 and miR-29a/b levels in AD were lower than control subjects[135-137]. Jain et al. also found a high expression of miR-27a-3p, miR-30a-5p and miR-34c in the cerebral spinal fluid of AD[138].
As for the regulating mechanisms, meta-analysis indicated that miR-129 is able to regulate synaptic plasticity and is present at low levels in brain regions of AD patients[139-140]. In addition, miRNAs also exert a wide range of modulation on APP processing and subsequent Aβ production. For example, miR-346 specifically targets the APP mRNA 5’-UTR to promote APP translation and Aβ production[141]. On the contrary, miR-455-3p regulates APP processing and protects against mutant APP-induced mitochondrial dysfunction and synaptic abnormalities in AD[142]. Moreover, miR-425, a neuronal-specific regulator is decreased in AD brain and promotes the amyloidogenic processing of APP, neuroinflammation, neuron loss, and cognitive impairment. In contrast, miR-425 supplementation ameliorated amyloid plaque-associated pathological changes and memory deficits[143].
Similar to miRNAs, piRNAs can also exhibit a different expression pattern in AD brain. For example, Qiu et al. identified 103 nominally differentially expressed piRNA from a total of 9,453 piRNAs in brains of AD patients compared with those of control subjects. The expression quantitative trait locus analysis further indicated that most of the 103 AD-related piRNAs were correlated with the genome-wide significant risk SNPs[144]. In another study, Jain et al. identified three piRNAs in the cerebral spinal fluid of AD patients, with a decreased expression level of piR-019324 but increased expression levels of piR-019949 and piR-020364. Interestingly, these piRNA alterations could predict conversion from MCI to AD with an AUC of 0.86. Moreover, the combined analysis of the piRNA profile with phosphorylated tau and Aβ42/40 ratio measurement was able to predict conversion from MCI to AD with a much higher AUC of 0.96[138].
Notably, epigenetic inheritance concerns the mechanisms that ensure the transmission of epigenetic marks from mother to daughter cells. Chromatin modifications and nuclear organization are candidates for epigenetic marks-whether they fulfill the criterion of heritability and what mechanisms ensure their propagation is an area of intensive research. The passage of the replication fork challenges genetic and epigenetic information. Depending on the nature of the epigenetic mark, its inheritance can be ensured in a replication-coupled manner or promptly that is separated from the disruptive event.
IMPACTS OF EXERCISE ON ALZHEIMER’S DISEASE
Cognitive decline induced by brain aging or various pathological conditions can be ameliorated by environmental enrichment (EE) exposure, a complex combination of multiple social, cognitive and physical stimulations[145,146]. EE improves behavior, cognition, and brain function in young senescence-accelerated-prone mice[147]. EE also restores age-related cognitive impairment in mice through transcriptomic mechanisms[148]. Chronic EE exposure relieves cognitive decline and synaptic function induced by prenatal inflammation in aged CD-1 mice[149]. More specifically for AD, EE exposure counteracts Alzheimer's neurovascular dysfunction in TgCRND8 mice[150]. Moreover, EE also prevents synaptic dysfunction induced by Aβ oligomer through miRNA-132 and hdac3 signaling pathways[151]. In humans, clinical trials indicated that enriched gardens improve the cognition and independence of nursing home residents with dementia[152]. Among all factors involved in EE, physical exercise may be the most interesting one and plays an important role in restoring impaired cognition and ameliorating the pathological progression of AD. In this section, we focus on physical exercise to discuss the beneficial impacts of exercise on cognition and AD pathologies.
Beneficial impacts of exercise on brain health and cognition
The benefits of physical exercise on brain health and cognitive function have also been separately investigated in both rodent and human subjects. The benefits might base on various mechanisms on anatomic, cellular and molecular levels. For example, voluntary wheel running reduced the anxiety level of mice, which was associated with changes in the brain fatty acid profile[153]. Treadmill training rescues anxiety and cognitive decline induced by chronic sleep deprivation in mice[154]. Choi et al. found that exercise provided cognitive benefits to 5 × FAD mouse model of AD by the induction of adult hippocampal neurogenesis and BDNF level[155]. Interestingly, administration of plasma from exercised mice transferred the effects of exercise on adult neurogenesis and cognition to sedentary aged mice[156]. In human subjects, 12 weeks of simultaneous exercise and cognitive training in visual reality elicit positive changes in brain volume, vascular resistance, memory, and executive function in cognitively normal older adults[157]. In addition, one clinical trial found that vigorous aerobic exercise training may improve specific aspects of cognitive function in individuals with traumatic brain injury[158].
Benefits of exercise on AD pathologies
Studies in humans and animal models suggest that exercise has protective effects against AD, but the underlying mechanisms still require further investigation. Previous studies have found that exercise can stimulate neurogenesis and ameliorate cognitive deficits in AD mouse models through FNDC5/irisin/BDNF signaling[155,159-162]. More interestingly, this pathway has also been involved in neuroprotection by regulating neuroinflammation, improving brain metabolism, and modulating APP processing[163-168], which may also contribute to the benefits of exercise on AD to rescue AD pathologies.
Previous studies have also documented other molecular signaling pathways through which exercise specifically rescues or prevents amyloid or tau pathologies in AD[169-173]. Glycogen synthase kinase-3 (GSK-3) has been confirmed as a key regulator of tau hyperphosphorylation and APP processing, promoting the progression of tauopathy and pathological Aβ aggregation in AD. One previous study found that 5 months of treadmill exercise inhibited GSK-3-dependent signaling, leading to decreased PS1 expression and APP phosphorylation, thereafter dramatically reduced Aβ production and tau phosphorylation in APP/PS1 mice[174]. In other studies, exercise activated PI3K/AKT, the upstream signaling of GSK-3, and inhibited GSK-3, thus mitigating the pathological changes of AD[175,176].
Recently, peroxisome proliferator-activated receptor-γ coactivator 1-α (PGC1-α), a transcription coactivator and the upstream signaling molecule of the FNDC5/irisin/BDNF pathway, has been reported to be activated by regular physical exercise and involved in the exercise-rescued cognitive deficits in AD[161]. Exercise epigenetically regulates PGC1α level through modulating methylation of the-260 nt in the PGC1α promoter. PGC-1α is closely associated with mitochondrial function and metabolism and is involved in various diseases including obesity, diabetes mellitus, cardiovascular disease, and neurological disorders. PGC-1α regulates the expression of mitochondrial antioxidant genes, and prevents oxidative stress and mitochondrial dysfunction. Abnormal PGC-1α function disrupts redox homeostasis and exacerbates inflammation, which results in neurodegeneration. During inflammation, the declined PGC-1α level leads to decreased mitochondrial antioxidant gene expression, induces oxidative stress, and promotes NF-κB activation. PGC-1α acts as an essential node connecting metabolic regulation, redox control, inflammation, and neuroprotection[177,178]. Interestingly, the benefits of PGC1-α stimulation on AD pathology can be attributed to its modulating activity on APP processing, especially β-site amyloid precursor protein cleaving enzyme-1 (BACE1), thus resulting in a decreased Aβ production and thereafter reduced pathological Aβ aggregation[179,180].
Notably, PGC1-α is also the substrate of Sirtuin-1 (SIRT1), an important protein controlling histone acetylation. Activation of SIRT1 signaling pathway, induced either pharmacologically (such as osmotin and resveratrol) or non-pharmacologically (photobiomodulation therapy), can rescue neurodegeneration via upregulating a disintegrin and metalloproteinase 10 (ADAM10) and down-regulating BACE1 activity[181-183]. Interestingly, exercise has been reported to recover the downregulated SIRT1 in 3xTg AD mice[184]. Moreover, Koo et al. showed that treadmill exercise promoted SIRT1 expression level, which subsequently caused the activation of ADAM10 by increasing the retinoic acid receptor-β and inhibiting Rho-associated kinase 1[185].
EPIGENETIC MECHANISMS FOR EXERCISE AGAINST ALZHEIMER’S PATHOLOGY
As mentioned above, increasing lines of evidence have indicated that exercise has positive impacts on brain health and cognitive function. However, the exact epigenetic mechanisms responsible are largely unknown. In this context, more and more studies have examined the epigenetic impacts of exercise on brain function under either physiological or pathological conditions [partially summarized in Table 2]. Although the experimental data that link exercise and epigenetics modulation in AD are still limited, these findings may open new avenues helping discover new targets and design innovative therapeutic strategies against AD. In this section, we mainly discussed the epigenetic mechanisms of exercise on AD.
Exercise-related epigenetic changes in the brain
Exercise | Animals | Brain region | Epigenetic changes | Reference |
Running wheel | Rats | Dentate gyrus | ↑ histone H3 phospho-acetylation | [189] |
Treadmill exercise | Rats | Hippocampus | ↓ HDAC activity; ↑ HAT activity; ↑ HAT/HDAC balance | [190] |
Running wheel | Rats | Hippocampus | ↓ DNA demethylation at Bdnf promoter IV; ↑ pMeCP2 levels; ↑ histone H3 acetylation; ↓ HDAC5 expression | [186] |
Treadmill exercise | Rats | Hippocampus | Adult rats: ↓ DNMT1 and DNMT3b; ↓ H3K9 methylation Aged rats: ↑ H3K9 methylation | [191] |
Running wheel | Mice | Hippocampus | ↑ histone H4K8 acetylation at Bdnf promoters I and IV | [187] |
Running wheel | Mice | Hippocampus and cerebellum | ↑ histone H3 acetylation in both regions; Cerebellum: ↑ HDAC2 and ↓ MeCP2, HDAC8 and DNMT1; Hippocampus: ↓ HDAC5, HDAC7, HDAC 8, DNMT1, DNMT3a, DNMT3b | [192] |
Treadmill exercise | Rats | Hippocampus | ↑ histone H4 acetylation in aged rats | [193] |
Treadmill exercise | Rats | Hippocampus | Exercise normalized stress-induced changes in histone H3 acetylation, HDAC5 and MeCP2 | [194] |
Treadmill exercise | Rats | Frontal cortex | ↑ HAT activity; ↓ HDAC activity | [195] |
Running wheel | Mice | Hippocampus | ↑ miR-28a-5p, miR-98a-5p, miR-148b-3p, miR-7a-5p and miR-15b-5p; ↓ miR-105, and miR-133b-3p | [196] |
Running wheel | Mice | Hippocampus | ↑ 20 miRNAs and ↓ 12 miRNAs | [197] |
Running wheel | Mice | Hippocampus | Exercise restored traumatic brain injury (TBI)-induced changes in miR-21 | [198] |
Running wheel | Mice | Hippocampus | Exercise attenuated the increased expression of miR-124 in a stress model | [199] |
Running wheel | Mice | Hippocampus | ↑ histone H3 acetylation at Bdnf promoters I, II, III, IV, VI and VII; ↓ HDAC5 expression in stressed mice | [200] |
Swimming exercise | Mice | Hippocampus | ↑ H3K9, H3K14, H4K5, H4K8 and H4K12 acetylation; ↑ CBP expression | [201] |
Running wheel | Mice | Basolateral amygdala | Exercise prevented the reduction in G9a histone methyltransferase expression induced by chronic stress; ↑ histone H3K9 dimethylation at oxytocin and vasopressin gene promoters | [202] |
Running wheel | Mice | Hippocampus | ↓ HDAC2 and HDAC3 expression; ↓ HDAC2 and HDAC3 occupancy at Bdnf promoters | [188] |
Treadmill exercise | Mice | hippocampus | ↑ HAT and HDAC activities | [203] |
Running wheel | Rats | Hippocampus and frontal cortex | DNA hypomethylation; ↑ Tet1 and ↓ Dnmt3b expression | [204] |
Treadmill exercise | Mice | Hippocampus | ↑ BDNF expression; ↓ HDAC activity, ↑ HAT/HDAC) | [205] |
Aerobic, acrobatic, resistance, or combined exercise modalities | Rats | Hippocampus | Aerobic and resistance modalities attenuated age-induced effects on hippocampal Bdnf promoter H3K4me3. Exercise modalities modify H3K9ac or H3K4me3 at the cFos promoter | [206] |
Forced running wheel | Mice | Hippocampus | Rescue the radiation-induced decrease of 5 hmC and BDNF expression | [207] |
Treadmill exercise | Rats | Motor cortex | ↑ 5mC and 5hmC; ↑ Tet1, Tet2, and Tet3 expression | [208] |
Treadmill exercise | Mice | Motor cortex | ↑ HDAC activity and acetylation level of histone H4 and H3 | [209] |
Treadmill exercise | Mice | Hippocampus and hypothalamus | ↑ level of N6-methyladenosine (m6A) | [210] |
Exercise-induced epigenetic alterations
Emerging experimental and clinical evidence from animals and humans has indicated that exercise can induce epigenetic alterations, which may contribute to its beneficial impacts on health. For instance, acute exercise in human subjects induced a global DNA hypomethylation, which leads to an increased expression of key metabolic and regulatory genes, including PGC-1α, peroxisome proliferator-activated receptor δ (PPAR-δ), mitochondrial transcription factor A (TFAM), and myocyte enhancer factor 2 (MEF2), in an exercise intensities-dependent manner[211]. In addition, short-term exercise also results in decreased methylation of the PGC-1α promoter in human skeletal muscle and induces a dramatic increase in PGC-1α gene expression together with postexercise alterations of lipid metabolism[212]. Although the exact molecular mechanisms of how exercise induces epigenetic modifications are still far from being clearly elucidated, one recent study has suggested a possible involvement of calcium signaling[211]. No clear correlations have been observed between gene expression and DNA methylation, suggesting the possible involvement of other epigenetic mechanisms in exercise-modulated gene expression. There is also no conclusive relationship between global DNA methylation patterns and AD pathologies. Therefore, attention has been shifted to the effect of gene-specific methylation on the risk of AD.
Besides DNA methylation, exercise also induces histone modifications. A previous study reported that exercise increases histone 3 (H3) serine phosphorylation in the skeletal muscle of both untrained and trained subjects[213]. In animal studies, swimming exercise in rats increased H3 K9/14 acetylation, a histone mark associated with transcriptional activation, at the glucose transporter type 4 (Glut4) promoter in the triceps muscle[214]. Consistent with the histone modifications observed in muscles, a number of studies have also found histone modifications in various brain regions in response to exercise. For instance, forced swimming training promotes H3 K14 acetylation and serine 10 phosphorylation in the dentate gyrus of rodents in a time-dependent manner[215]. Moreover, voluntary exercise increases H3 acetylation at the BDNF promoter in the hippocampus of rats[216].
Exercise, epigenetic modulation on BDNF, and Alzheimer’s disease
More and more robust experimental evidence supports the positive impacts of physical exercise on psychiatric and neurological disorders, and epigenetic regulation of the BDNF gene has been recognized as an important biological mechanism by which exercise ameliorates neurological disorders[217,218], including AD. For example, peripheral levels of BDNF have been associated with cognitive function and hippocampal size in human subjects after exercise training[219,220]. Consistently, animal studies have also suggested the involvement of BDNF in the exercise-modulated expression of energy metabolism- and neurocognitive plasticity-related proteins in the hippocampus[221-222]. Specifically for AD, recent studies have identified the involvement of BDNF signaling in AD pathogenesis and the cognitive benefits of exercise on AD. Choi found that exercise provided cognitive benefit to 5 × FAD model mice of AD by inducing adult hippocampal neurogenesis and elevating BDNF levels[155]. In addition, chronic aerobic exercise can ameliorate Aβ-induced AD-like phenotype in rats through BDNF signaling[223].
As for the mechanisms involved in the BDNF-mediated benefits of exercise on AD, previous studies have indicated that exercise can modulate the activities of α-secretase and BACE1 through BDNF-mediated mechanisms, thereafter regulate APP processing and reduce Aβ production[224,225]. Moreover, exercise-induced epigenetic modifications of the BDNF gene, such as acetylation and methylation, have also been reported to play important roles[186-188]. For example, thirty days of voluntary running wheel training in mice induced hippocampal HDAC2 reduction, accompanied by the decreased interaction between HDAC2 and BDNF promoter I, leading to an increased BDNF expression[188]. Interestingly, the increased BDNF can further promote nitrosylation of HDAC2, resulting in increased histone acetylation in those BDNF target genes[226]. Despite the exercise-induced BDNF gene acetylation, Gomez-Pinilla et al. found that 7 days of running wheel exercise in rats reduced the level of DNA methylation in BDNF promoter IV in the hippocampus, accompanied by an elevated methyl-CpG-binding protein 2 (MeCP2) and increased BDNF mRNA and protein levels[186]. The elevated BDNF expression and cognitive improvement induced by exercise can be attenuated by the inhibition of Calcium-calmodulin-dependent protein kinase II[227,228].
Much more interestingly, BDNF transcription has been reported to be regulated by SIRT1-dependent deacetylation of MeCP2[229]. As mentioned above, SIRT1, beyond its complicated impacts on aging, stress tolerance, and metabolism, exerts key regulating activity on synaptic plasticity and memory formation. SIRT1-dependent deacetylation of MeCP2 permits its release from the methylated CpG site located in BDNF promoter IV and results in an increased BDNF transcription[229]. Moreover, SIRT1Δex4 mice exhibit significantly higher recruitment of MeCP2 on BDNF promoter IV, which was associated with decreased BDNF expression in hippocampus[229]. Consistent with these findings, treadmill running exercise in rats was found to increase SIRT1 activity in the hippocampus, together with increased BDNF and decreased apoptotic index[230]. In addition, treadmill running in ICR mice can also elevate SIRT1 and PGC1-α expression levels in various brain regions including cortex, hippocampus, hypothalamus, and midbrain, and[231]. Furthermore, the suppressed SIRT1 in the cerebral cortex of 3xTgAD mice was attenuated by treadmill exercise training[184].
As mentioned above, the exercise-modulated SIRT1 impacts the function of not only BDNF but also other enzymes involved in APP metabolism, including ADAM10 and BACE1, through epigenetic mechanisms. Activation of SIRT1 induces PGC-1α deacetylation, leading to the decreased BACE1 transcription and consequently reduction of Aβ production. SIRT1 also deacetylates and coactivates the retinoic acid receptor β, a known regulator of ADAM10 transcription, to promote the ADAM10-mediated non-amyloidogenic processing of APP[232]. Moreover, SIRT1 activation suppresses tau acetylation on K174 and reduces pathological tau propagation in the mouse models of tauopathy[233]. Taken together, exercise-induced SIRT1 activation may promote neurogeneration and plasticity and suppress the progression of AD pathologies through epigenetic modifications on targeted genes.
Exercise, microRNA and Alzheimer’s disease
miRNAs are important epigenetic modulating molecules involved in the beneficial impacts of exercise on AD. For example, miR-29 has been reported to be decreased in either AD patients[137] or transgenic AD model mice[234], and correlated with increased BACE1 levels. Progressive weighted wheel running in 3xTg-AD mice significantly increased this declined miR-29 level in the hippocampus and consequently reduced BACE1 level and Aβ burden[234]. Moreover, miR-34a has been reported to be upregulated in APP/PS1 transgenic mice[235,236]. Overexpression of miR-34a depresses ADAM10 expression and induces rapid cognitive impairment and AD-like pathologies[237], whereas knockout of miR-34a has been reported to modulate APP processing via inhibiting γ-secretase thereafter rescues cognitive deficits of APP/PS1 mice[238]. Interestingly, treadmill training can elevate miR-34a levels in mouse hippocampus[238]. Consistently, Kou et al. also found that swimming can attenuate autophagy dysfunction and abnormal mitochondrial dynamics via downregulating miR-34a, thus improving pathologies in aging-related diseases including AD[239]. Worth noting, miR-34a also targets and inhibits SIRT1[240,241], which is also involved in the exercise-induced AD improvements via PGC1-α/FNDC5/irisin/BDNF signaling, as described above.
MiR-132, another sncRNA with pivotal activity in neuronal development, structure and function, has been reported to regulate dendritic spine formation and maturation, so as to be involved in learning and memory. However, the modulating activity of miR-132 on cognition is controversial, because this effect is very dependent on its levels under physiological or pathological conditions. Lower[242] or higher[243] levels of miR-132 may be detrimental to cognition, while only moderate levels are beneficial[244]. It has been reported that acute intermittent exercise rapidly elevates circulating levels of miR-132 in healthy males[245]. In contrast, voluntary running wheel training can suppress the elevated hippocampal miR-132 level and ameliorate cognitive impairment in SAMP8 mice[246]. Consistently, swimming exercise reduced the increased miR-132 level in an ovariectomized rat model[247]. In addition, despite the direct modulating effects of miR-132 on cognition, previous studies have found an inhibiting effect of miR-132 on GSK-3β expression, leading to an ameliorated tau phosphorylation in hyperglycemia or chronic cerebral hypoperfusion animal model[248,249]. Moreover, miR-132-3p alleviates impairments of learning and memory abilities in AD-like homocysteine rat models by modulating the HNRNPU/BACE1 axis[250]. miR-132/212 deficiency led to cognitive impairment and tau hyperphosphorylation and aggregation in miR-132/212 knockout mice[251], promoting Aβ production and plaque formation in 3xTg AD mice[252]. These findings suggested that miR-132 is correlated with Aβ/tau pathologies of AD, which may provide further evidence supporting the positive impacts of exercise on AD through miR-132-related epigenetic mechanisms. Interestingly, the ameliorating effects of miR-132 on AD pathologies are also correlated with exercise-related SIRT1 signaling, suggesting a crosslink role of SIRT1 in miRNAs-mediated regulating effects against AD pathologies.
A previous study found that exercise reduces miR-146a level and increases miR-223 level in circulation in young healthy males[253]. As an NF-κB-sensitive miRNA, miR-146a has been reported to be elevated in AD and be closely associated with the proinflammatory state of AD[254-256]. This miRNA is correlated with the severity of AD[257] and involved in the progression of MCI to AD[258]. miR-223 is also a downstream molecule of NF-κB and is correlated with Nod-like receptor protein 3 inflammasome activation in AD[259,260]. miR-223 is downregulated in AD, either in serum of AD patients[261] or in AD cell models[262]. In turn, the lack of miR-223 leads to hippocampal-dependent contextual memory deficits and neuronal cell death[263].
CONCLUSION
Exercise plays important roles in brain health and cognition through various mechanisms, including elevated neurotrophins level, improved neurogenesis and neuroplasticity, restored angiogenesis and autophagy, and reduced neuroinflammation. In addition, exercise is also a vitally instrumental and daily-life remedy for reducing the susceptibility of brain to a wide range of neurological and neurodegenerative conditions, including AD. Recently, accumulating scientific evidence demonstrated the capacity of exercise to modulate genes and their protein products in the form of epigenomic manifestations. This promising impact of epigenetic mechanisms to regulate neuronal survival and plasticity has fundamental values to control or rescue pathological changes of AD [Figure 1].
Figure 1. Exercise ameliorates Alzheimer’s pathologies and cognition decline through epigenetic mechanisms. DNA and histone modifications and non-coding RNA profile alterations induced by various adverse environmental factors may result in elevated Aβ production, tau phosphorylation, neuroinflammation and neurodegeneration, and consequently participates in the cognitive decline and the pathogenesis of Alzheimer’s disease. In contrast, regular physical exercise can ameliorate these negative impacts caused by an aversive environment also through epigenetic mechanisms, thereafter directly or indirectly improving cognitive function and rescuing Alzheimer’s pathologies.
Following much deeper investigations in the future to reveal the exact spatial-temporal epigenetic modulating network by which exercise influences the expression or functions of AD-related genes and protein products, it will be clearer that epigenetic modifications by either pharmacological or
DECLARATIONS
Acknowledgments
The author would like to thank Professor Che Wang from Liaoning Normal University for her discussion and constructive comments during manuscript preparation.
Authors’ contributions
The author contributed solely to the article.
Availability of data and materials
Not applicable.
Financial support and sponsorship
None.
Conflicts of interest
The author declared that there are no conflicts of interest.
Ethical approval and consent to participate
Not applicable.
Consent for publication
Not applicable.
Copyright
© The Author(s) 2023.
REFERENCES
1. Cunningham C, O' Sullivan R, Caserotti P, Tully MA. Consequences of physical inactivity in older adults: a systematic review of reviews and meta-analyses. Scand J Med Sci Sports 2020;30:816-27.
2. Patterson SL, Trupin L, Yazdany J, et al. Physical inactivity and incident depression in a multiracial, multiethnic systemic lupus erythematosus cohort. Arthritis Care Res 2022;74:1098-104.
3. Hamer M, Chida Y. Physical activity and risk of neurodegenerative disease: a systematic review of prospective evidence. Psychol Med 2009;39:3-11.
4. Santiago JA, Quinn JP, Potashkin JA. Physical activity rewires the human brain against neurodegeneration. Int J Mol Sci 2022;23:6223.
5. Sujkowski A, Hong L, Wessells RJ, Todi SV. The protective role of exercise against age-related neurodegeneration. Ageing Res Rev 2022;74:101543.
6. López-Ortiz S, Lista S, Valenzuela PL, et al. Effects of physical activity and exercise interventions on Alzheimer’s disease: an umbrella review of existing meta-analyses. J Neurol 2023;270:711-25.
7. Gubert C, Hannan AJ. Exercise mimetics: harnessing the therapeutic effects of physical activity. Nat Rev Drug Discov 2021;20:862-79.
8. Freitas GB, Lourenco MV, De Felice FG. Protective actions of exercise-related FNDC5/Irisin in memory and Alzheimer's disease. J Neurochem 2020;155:602-11.
9. Gholamnezhad Z, Boskabady MH, Jahangiri Z. Exercise and Dementia. In: Xiao J, editor. Physical exercise for human health. Singapore: Springer; 2020. pp. 303-15.
10. da Costa Daniele TM, de Bruin PFC, de Matos RS, et al. Exercise effects on brain and behavior in healthy mice, Alzheimer's disease and Parkinson's disease model-A systematic review and meta-analysis. Behav Brain Res 2020;383:112488.
11. Sawan C, Vaissière T, Murr R, Herceg Z. Epigenetic drivers and genetic passengers on the road to cancer. Mutat Res 2008;642:1-13.
12. Turek-Plewa J, Jagodziński PP. The role of mammalian DNA methyltransferases in the regulation of gene expression. Cell Mol Biol Lett; 2005.10.631-47.
13. Lyko F. The DNA methyltransferase family: a versatile toolkit for epigenetic regulation. Nat Rev Genet 2018;19:81-92.
14. Wu X, Zhang Y. TET-mediated active DNA demethylation: mechanism, function and beyond. Nat Rev Genet 2017;18:517-34.
15. Loenarz C, Schofield CJ. Oxygenase catalyzed 5-methylcytosine hydroxylation. Chem Biol 2009;16:580-3.
16. Inoue A, Zhang Y. Replication-dependent loss of 5-hydroxymethylcytosine in mouse preimplantation embryos. Science 2011;334:194.
17. Ito S, Shen L, Dai Q, et al. Tet proteins can convert 5-methylcytosine to 5-formylcytosine and 5-carboxylcytosine. Science 2011;333:1300-3.
18. Rea S, Eisenhaber F, O'Carroll D, et al. Regulation of chromatin structure by site-specific histone H3 methyltransferases. Nature 2000;406:593-9.
20. Earls LR, Westmoreland JJ, Zakharenko SS. Non-coding RNA regulation of synaptic plasticity and memory: implications for aging. Ageing Res Rev 2014;17:34-42.
21. Feng J, Zhou Y, Campbell SL, et al. Dnmt1 and dnmt3a maintain DNA methylation and regulate synaptic function in adult forebrain neurons. Nat Neurosci 2010;13:423-30.
22. Maddox SA, Watts CS, Schafe GE. DNA methyltransferase activity is required for memory-related neural plasticity in the lateral amygdala. Neurobiol Learn Mem 2014;107:93-100.
23. Miller CA, Sweatt JD. Covalent modification of DNA regulates memory formation. Neuron 2007;53:857-69.
24. Lubin FD, Roth TL, Sweatt JD. Epigenetic regulation of BDNF gene transcription in the consolidation of fear memory. J Neurosci 2008;28:10576-86.
25. Levenson JM, Roth TL, Lubin FD, et al. Evidence that DNA (cytosine-5) methyltransferase regulates synaptic plasticity in the hippocampus. J Biol Chem 2006;281:15763-73.
26. Argyrousi EK, de Nijs L, Lagatta DC, et al. Effects of DNA methyltransferase inhibition on pattern separation performance in mice. Neurobiol Learn Mem 2019;159:6-15.
27. Miller CA, Gavin CF, White JA, et al. Cortical DNA methylation maintains remote memory. Nat Neurosci 2010;13:664-6.
28. Taylor RM, Smith R, Collins CE, et al. Global DNA methylation and cognitive and behavioral outcomes at 4 years of age: A cross-sectional study. Brain Behav 2020;10:e01579.
29. Schiepers OJ, van Boxtel MP, de Groot RH, et al. DNA methylation and cognitive functioning in healthy older adults. Br J Nutr 2012;107:744-8.
30. Collins BE, Greer CB, Coleman BC, Sweatt JD. Histone H3 lysine K4 methylation and its role in learning and memory. Epigenetics Chromatin 2019;12:7.
31. Keiser AA, Wood MA. Examining the contribution of histone modification to sex differences in learning and memory. Learn Mem 2019;26:318-31.
32. Geng H, Chen H, Wang H, Wang L. The histone modifications of neuronal plasticity. Neural Plast 2021;2021:6690523.
33. Maity S, Farrell K, Navabpour S, Narayanan SN, Jarome TJ. Epigenetic mechanisms in memory and cognitive decline associated with aging and Alzheimer’s disease. Int J Mol Sci 2021;22:12280.
34. Gupta S, Kim SY, Artis S, et al. Histone methylation regulates memory formation. J Neurosci 2010;30:3589-99.
35. Jakovcevski M, Ruan H, Shen EY, et al. Neuronal kmt2a/Mll1 histone methyltransferase is essential for prefrontal synaptic plasticity and working memory. J Neurosci 2015;35:5097-108.
36. Webb WM, Sanchez RG, Perez G, et al. Dynamic association of epigenetic H3K4me3 and DNA 5hmC marks in the dorsal hippocampus and anterior cingulate cortex following reactivation of a fear memory. Neurobiol Learn Mem 2017;142:66-78.
37. Jarome TJ, Perez GA, Hauser RM, Hatch KM, Lubin FD. EZH2 Methyltransferase activity controls pten expression and mtor signaling during fear memory reconsolidation. J Neurosci 2018;38:7635-48.
38. Gupta-Agarwal S, Franklin AV, Deramus T, et al. G9a/GLP histone lysine dimethyltransferase complex activity in the hippocampus and the entorhinal cortex is required for gene activation and silencing during memory consolidation. J Neurosci 2012;32:5440-53.
39. Gupta-Agarwal S, Jarome TJ, Fernandez J, Lubin FD. NMDA receptor- and ERK-dependent histone methylation changes in the lateral amygdala bidirectionally regulate fear memory formation. Learn Mem 2014;21:351-62.
40. Peixoto L, Abel T. The role of histone acetylation in memory formation and cognitive impairments. Neuropsychopharmacology 2013;38:62-76.
42. Alarcón JM, Malleret G, Touzani K, et al. Chromatin acetylation, memory, and LTP are impaired in CBP+/- mice: a model for the cognitive deficit in Rubinstein-Taybi syndrome and its amelioration. Neuron 2004;42:947-59.
43. Maurice T, Duclot F, Meunier J, et al. Altered memory capacities and response to stress in p300/CBP-associated factor (PCAF) histone acetylase knockout mice. Neuropsychopharmacology 2008;33:1584-602.
44. Guan JS, Haggarty SJ, Giacometti E, et al. HDAC2 negatively regulates memory formation and synaptic plasticity. Nature 2009;459:55-60.
45. Wang WH, Cheng LC, Pan FY, et al. Intracellular trafficking of histone deacetylase 4 regulates long-term memory formation. Anat Reck 2011;294:1025-34.
46. Sando R 3rd, Gounko N, Pieraut S, Liao L, Yates J 3rd, Maximov A. HDAC4 governs a transcriptional program essential for synaptic plasticity and memory. Cell 2012;151:821-34.
47. Kim MS, Akhtar MW, Adachi M, et al. An essential role for histone deacetylase 4 in synaptic plasticity and memory formation. J Neurosci 2012;32:10879-86.
48. Agis-Balboa RC, Pavelka Z, Kerimoglu C, Fischer A. Loss of HDAC5 impairs memory function: implications for Alzheimer’s disease. J Alzheimers Dis 2013;33:35-44.
49. Gräff J, Woldemichael BT, Berchtold D, Dewarrat G, Mansuy IM. Dynamic histone marks in the hippocampus and cortex facilitate memory consolidation. Nat Commun 2012;3:991.
50. Chwang WB, O'Riordan KJ, Levenson JM, Sweatt JD. ERK/MAPK regulates hippocampal histone phosphorylation following contextual fear conditioning. Learn Mem 2006;13:322-8.
51. Chwang WB, Arthur JS, Schumacher A, Sweatt JD. The nuclear kinase mitogen- and stress-activated protein kinase 1 regulates hippocampal chromatin remodeling in memory formation. J Neurosci 2007;27:12732-42.
52. Yamamoto Y, Verma UN, Prajapati S, Kwak YT, Gaynor RB. Histone H3 phosphorylation by IKK-alpha is critical for cytokine-induced gene expression. Nature 2003;423:655-9.
53. Lubin FD, Sweatt JD. The IkappaB kinase regulates chromatin structure during reconsolidation of conditioned fear memories. Neuron 2007;55:942-57.
54. Jarome TJ, Perez GA, Webb WM, et al. Ubiquitination of histone H2B by proteasome subunit rpt6 controls histone methylation chromatin dynamics during memory formation. Biol Psychiatry 2021;89:1176-87.
55. Miller CA, Campbell SL, Sweatt JD. DNA methylation and histone acetylation work in concert to regulate memory formation and synaptic plasticity. Neurobiol Learn Mem 2008;89:599-603.
56. Koshibu K, Gräff J, Beullens M, et al. Protein phosphatase 1 regulates the histone code for long-term memory. J Neurosci 2009;29:13079-89.
57. Koshibu K, Gräff J, Mansuy IM. Nuclear protein phosphatase-1: an epigenetic regulator of fear memory and amygdala long-term potentiation. Neuroscience 2011;173:30-6.
58. Gräff J, Tsai LH. Histone acetylation: molecular mnemonics on the chromatin. Nat Rev Neurosci 2013;14:97-111.
59. Grinman E, Espadas I, Puthanveettil SV. Emerging roles for long noncoding RNAs in learning, memory and associated disorders. Neurobiol Learn Mem 2019;163:107034.
60. Woldemichael BT, Mansuy IM. Micro-RNAs in cognition and cognitive disorders: Potential for novel biomarkers and therapeutics. Biochem Pharmacol 2016;104:1-7.
61. Barry G. Integrating the roles of long and small non-coding RNA in brain function and disease. Mol Psychiatry 2014;19:410-6.
62. Im HI, Kenny PJ. MicroRNAs in neuronal function and dysfunction. Trends Neurosci 2012; 35:325-334.
63. Gao J, Wang WY, Mao YW, et al. A novel pathway regulates memory and plasticity via SIRT1 and miR-134. Nature 2010;466:1105-9.
64. Daswani R, Gilardi C, Soutschek M, et al. MicroRNA-138 controls hippocampal interneuron function and short-term memory in mice. Elife 2022;11:e74056.
65. Landry CD, Kandel ER, Rajasethupathy P. New mechanisms in memory storage: piRNAs and epigenetics. Trends Neurosci 2013; 36:535-542.
66. Rajasethupathy P, Antonov I, Sheridan R, et al. A role for neuronal piRNAs in the epigenetic control of memory-related synaptic plasticity. Cell 2012;149:693-707.
67. Leighton LJ, Wei W, Marshall PR, et al. Disrupting the hippocampal Piwi pathway enhances contextual fear memory in mice. Neurobiol Learn Mem 2019;161:202-9.
68. Derrien T, Johnson R, Bussotti G, et al. The GENCODE v7 catalog of human long noncoding RNAs: analysis of their gene structure, evolution, and expression. Genome Res 2012;22:1775-89.
69. Spadaro PA, Flavell CR, Widagdo J, et al. Long noncoding RNA-directed epigenetic regulation of gene expression is associated with anxiety-like behavior in mice. Biol Psychiatry 2015;78:848-59.
70. Wen X, Han XR, Wang YJ, et al. Down-regulated long non-coding RNA ANRIL restores the learning and memory abilities and rescues hippocampal pyramidal neurons from apoptosis in streptozotocin-induced diabetic rats via the NF-κB signaling pathway. J Cell Biochem 2018;119:5821-5833.
71. Butler AA, Johnston DR, Kaur S, Lubin FD. Long noncoding RNA NEAT1 mediates neuronal histone methylation and age-related memory impairment. Sci Signal 2019:12.
72. Sarangdhar MA, Chaubey D, Bhatt A, et al. A novel long non-coding RNA, durga modulates dendrite density and expression of kalirin in zebrafish. Front Mol Neurosci 2017;10:95.
74. Nikolac Perkovic M, Videtic Paska A, Konjevod M, et al. Epigenetics of Alzheimer's disease. Biomolecules 2021;11:195.
75. Qazi TJ, Quan Z, Mir A, Qing H. Epigenetics in Alzheimer's disease: perspective of dna methylation. Mol Neurobiol 2018;55:1026-1044.
76. Lord J, Cruchaga C. The epigenetic landscape of Alzheimer's disease. Nat Neurosci 2014;17:1138-40.
77. Migliore L, Coppedè F. Gene-environment interactions in Alzheimer disease: the emerging role of epigenetics. Nat Rev Neurol 2022;18:643-60.
78. Gasparoni G, Bultmann S, Lutsik P, et al. DNA methylation analysis on purified neurons and glia dissects age and Alzheimer's disease-specific changes in the human cortex. Epigenetics Chromatin 2018;11:41.
79. Lardenoije R, Roubroeks JAY, Pishva E, et al. Alzheimer's disease-associated (hydroxy)methylomic changes in the brain and blood. Clin Epigenetics 2019;11:164.
80. Haertle L, Müller T, Lardenoije R, et al. Methylomic profiling in trisomy 21 identifies cognition- and Alzheimer's disease-related dysregulation. Clin Epigenetics 2019;11:195.
81. Tohgi H, Utsugisawa K, Nagane Y, Yoshimura M, Genda Y, Ukitsu M. Reduction with age in methylcytosine in the promoter region -224 approximately -101 of the amyloid precursor protein gene in autopsy human cortex. Brain Res Mol Brain Res 1999;70:288-92.
82. West RL, Lee JM, Maroun LE. Hypomethylation of the amyloid precursor protein gene in the brain of an Alzheimer's disease patient. J Mol Neurosci 1995;6:141-6.
83. Li P, Marshall L, Oh G, et al. Epigenetic dysregulation of enhancers in neurons is associated with Alzheimer's disease pathology and cognitive symptoms. Nat Commun 2019;10:2246.
84. Foraker J, Millard SP, Leong L, et al. The APOE gene is differentially methylated in Alzheimer's disease. J Alzheimers Dis 2015;48:745-55.
85. Ferri E, Arosio B, D'Addario C, et al. Gene promoter methylation and expression of Pin1 differ between patients with frontotemporal dementia and Alzheimer's disease. J Neurol Sci 2016;362:283-6.
86. Nicolia V, Fuso A, Cavallaro RA, Di Luzio A, Scarpa S. B vitamin deficiency promotes tau phosphorylation through regulation of GSK3beta and PP2A. J Alzheimers Dis 2010;19:895-907.
87. Smith AR, Smith RG, Burrage J, et al. A cross-brain regions study of ANK1 DNA methylation in different neurodegenerative diseases. Neurobiol Aging 2019;74:70-6.
88. Pathak GA, Silzer TK, Sun J, et al. Genome-wide methylation of mild cognitive impairment in mexican americans highlights genes involved in synaptic transport, Alzheimer’s disease-precursor phenotypes, and metabolic morbidities. J Alzheimers Dis 2019;72:733-49.
89. Mano T, Nagata K, Nonaka T, et al. Neuron-specific methylome analysis reveals epigenetic regulation and tau-related dysfunction of BRCA1 in Alzheimer’s disease. Proc Natl Acad Sci USA 2017;114:E9645-54.
90. Semick SA, Bharadwaj RA, Collado-Torres L, et al. Integrated DNA methylation and gene expression profiling across multiple brain regions implicate novel genes in Alzheimer’s disease. Acta Neuropathol 2019;137:557-69.
91. Smith AR, Smith RG, Pishva E, et al. Parallel profiling of DNA methylation and hydroxymethylation highlights neuropathology-associated epigenetic variation in Alzheimer's disease. Clin Epigenetics 2019;11:52.
92. Hernández HG, Sandoval-Hernández AG, Garrido-Gil P, et al. Alzheimer’s disease DNA methylome of pyramidal layers in frontal cortex: laser-assisted microdissection study. Epigenomics 2018;10:1365-82.
93. Kaut O, Ramirez A, Pieper H, Schmitt I, Jessen F, Wüllner U. DNA methylation of the TNF-α promoter region in peripheral blood monocytes and the cortex of human Alzheimer's disease patients. Dement Geriatr Cogn Disord 2014;38:10-5.
94. Smith RG, Hannon E, De Jager PL, et al. Elevated DNA methylation across a 48-kb region spanning the HOXA gene cluster is associated with Alzheimer's disease neuropathology. Alzheimers Dement 2018;14:1580-8.
95. Bustos FJ, Ampuero E, Jury N, et al. Epigenetic editing of the Dlg4/PSD95 gene improves cognition in aged and Alzheimer’s disease mice. Brain 2017;140:3252-68.
96. Sanchez-Mut JV, Heyn H, Silva BA, et al. PM20D1 is a quantitative trait locus associated with Alzheimer’s disease. Nat Med 2018;24:598-603.
97. Marques SC, Lemos R, Ferreiro E, et al. Epigenetic regulation of BACE1 in Alzheimer’s disease patients and in transgenic mice. Neuroscience 2012;220:256-66.
98. Lu X, Deng Y, Yu D, et al. Histone acetyltransferase p300 mediates histone acetylation of PS1 and BACE1 in a cellular model of Alzheimer’s disease. PLoS One 2014;9:e103067.
99. Kumar A, Thakur MK. Epigenetic regulation of presenilin 1 and 2 in the cerebral cortex of mice during development. Dev Neurobiol 2015;75:1165-73.
100. Marzi SJ, Leung SK, Ribarska T, et al. A histone acetylome-wide association study of Alzheimer’s disease identifies disease-associated H3K27ac differences in the entorhinal cortex. Nat Neurosci 2018;21:1618-27.
101. Nott A, Holtman IR, Coufal NG, et al. Brain cell type-specific enhancer-promoter interactome maps and disease-risk association. Science 2019;366:1134-9.
102. Fetahu IS, Ma D, Rabidou K, et al. Epigenetic signatures of methylated DNA cytosine in Alzheimer’s disease. Sci Adv 2019;5:eaaw2880.
103. Wood H. Alzheimer disease: AD-susceptible brain regions exhibit altered DNA methylation. Nat Rev Neurol 2014;10:548.
104. Lin HC, Hsieh HM, Chen YH, Hu ML. S-Adenosylhomocysteine increases beta-amyloid formation in BV-2 microglial cells by increased expressions of beta-amyloid precursor protein and presenilin 1 and by hypomethylation of these gene promoters. Neurotoxicology 2009;30:622-7.
105. Di Francesco A, Arosio B, Falconi A, et al. Global changes in DNA methylation in Alzheimer’s disease peripheral blood mononuclear cells. Brain Behav Immun 2015;45:139-44.
106. De Jager PL, Srivastava G, Lunnon K, et al. Alzheimer’s disease: early alterations in brain DNA methylation at ANK1, BIN1, RHBDF2 and other loci. Nat Neurosci 2014; 17:1156-63.
107. Lunnon K, Smith R, Hannon E, et al. Methylomic profiling implicates cortical deregulation of ANK1 in Alzheimer's disease. Nat Neurosci 2014;17:1164-70.
108. Chouliaras L, Mastroeni D, Delvaux E, et al. Consistent decrease in global DNA methylation and hydroxymethylation in the hippocampus of Alzheimer’s disease patients. Neurobiol Aging 2013;34:2091-9.
109. Tong Z, Han C, Qiang M, et al. Age-related formaldehyde interferes with DNA methyltransferase function, causing memory loss in Alzheimer's disease. Neurobiol Aging 2015;36:100-10.
110. Mastroeni D, McKee A, Grover A, Rogers J, Coleman PD. Epigenetic differences in cortical neurons from a pair of monozygotic twins discordant for Alzheimer’s disease. PLoS One 2009;4:e6617.
111. Coppieters N, Dieriks BV, Lill C, Faull RL, Curtis MA, Dragunow M. Global changes in DNA methylation and hydroxymethylation in Alzheimer’s disease human brain. Neurobiol Aging 2014;35:1334-44.
112. Bakulski KM, Dolinoy DC, Sartor MA, et al. Genome-wide DNA methylation differences between late-onset Alzheimer's disease and cognitively normal controls in human frontal cortex. J Alzheimers Dis 2012;29:571-88.
113. Nativio R, Lan Y, Donahue G, et al. An integrated multi-omics approach identifies epigenetic alterations associated with Alzheimer's disease. Nat Genet 2020;52:1024-1035.
114. Lu T, Ang CE, Zhuang X. Spatially resolved epigenomic profiling of single cells in complex tissues. Cell 2022;185:4448-4464.e17.
115. Francis YI, Fà M, Ashraf H, et al. Dysregulation of histone acetylation in the APP/PS1 mouse model of Alzheimer's disease. J Alzheimers Dis 2009;18:131-9.
116. Ricobaraza A, Cuadrado-Tejedor M, Pérez-Mediavilla A, Frechilla D, Del Río J, García-Osta A. Phenylbutyrate ameliorates cognitive deficit and reduces tau pathology in an Alzheimer's disease mouse model. Neuropsychopharmacology 2009;34:1721-32.
117. Kim C, Choi H, Jung ES, et al. HDAC6 inhibitor blocks amyloid beta-induced impairment of mitochondrial transport in hippocampal neurons. PLoS One 2012;7:e42983.
118. Onishi T, Maeda R, Terada M, et al. A novel orally active HDAC6 inhibitor T-518 shows a therapeutic potential for Alzheimer's disease and tauopathy in mice. Sci Rep 2021;11:15423.
119. Gräff J, Rei D, Guan JS, et al. An epigenetic blockade of cognitive functions in the neurodegenerating brain. Nature 2012;483:222-6.
120. Zhang K, Schrag M, Crofton A, Trivedi R, Vinters H, Kirsch W. Targeted proteomics for quantification of histone acetylation in Alzheimer’s disease. Proteomics 2012;12:1261-8.
121. Anderson KW, Turko IV. Histone post-translational modifications in frontal cortex from human donors with Alzheimer’s disease. Clin Proteomics 2015;12:26.
122. Lithner CU, Lacor PN, Zhao WQ, et al. Disruption of neocortical histone H3 homeostasis by soluble Aβ: implications for Alzheimer’s disease. Neurobiol Aging 2013;34:2081-90.
123. Persico G, Casciaro F, Amatori S, et al. Histone H3 lysine 4 and 27 trimethylation landscape of human Alzheimer's disease. Cells 2022;11:734.
124. Zheng Y, Liu A, Wang ZJ, et al. Inhibition of EHMT1/2 rescues synaptic and cognitive functions for Alzheimer's disease. Brain 2019;142:787-807.
125. Cao Q, Wang W, Williams JB, Yang F, Wang ZJ, Yan Z. Targeting histone K4 trimethylation for treatment of cognitive and synaptic deficits in mouse models of Alzheimer's disease. Sci Adv 2020;6:eabc8096.
127. Wang E, Lemos Duarte M, Rothman LE, Cai D, Zhang B. Non-coding RNAs in Alzheimer's disease: perspectives from omics studies. Hum Mol Genet 2022;31:R54-R61.
128. Lauretti E, Dabrowski K, Praticò D. The neurobiology of non-coding RNAs and Alzheimer's disease pathogenesis: Pathways, mechanisms and translational opportunities. Ageing Res Rev 2021;71:101425.
129. Yu CC, Jiang T, Yang AF, Du YJ, Wu M, Kong LH. Epigenetic modulation on tau phosphorylation in Alzheimer's disease. Neural Plast 2019;2019:6856327.
130. Lan Z, Chen Y, Jin J, Xu Y, Zhu X. Long non-coding RNA: insight into mechanisms of Alzheimer's disease. Front Mol Neurosci 2022;14:821002.
131. Praticò D. The functional role of microRNAs in the pathogenesis of tauopathy. Cells 2020;9:2262.
132. Walgrave H, Zhou L, De Strooper B, Salta E. The promise of microRNA-based therapies in Alzheimer's disease: challenges and perspectives. Mol Neurodegener 2021;16:76.
133. Kim J, Inoue K, Ishii J, et al. A MicroRNA feedback circuit in midbrain dopamine neurons. Science 2007;317:1220-4.
134. Islam MR, Kaurani L, Berulava T, et al. A microRNA signature that correlates with cognition and is a target against cognitive decline. EMBO Mol Med 2021;13:e13659.
135. Manzine PR, Pelucchi S, Horst MA, et al. microRNA 221 targets ADAM10 mRNA and is downregulated in Alzheimer's disease. J Alzheimers Dis 2018;61:113-123.
136. Lau P, Bossers K, Janky R, et al. Alteration of the microRNA network during the progression of Alzheimer's disease. EMBO Mol Med 2013;5:1613-34.
137. Geekiyanage H, Jicha GA, Nelson PT, Chan C. Blood serum miRNA: non-invasive biomarkers for Alzheimer's disease. Exp Neurol 2012;235:491-6.
138. Jain G, Stuendl A, Rao P, et al. A combined miRNA-piRNA signature to detect Alzheimer's disease. Transl Psychiatry 2019;9:250.
139. Hosseinian S, Arefian E, Rakhsh-Khorshid H, et al. A meta-analysis of gene expression data highlights synaptic dysfunction in the hippocampus of brains with Alzheimer's disease. Sci Rep 2020;10:8384.
140. Dobricic V, Schilling M, Schulz J, et al. Differential microRNA expression analyses across two brain regions in Alzheimer's disease. Transl Psychiatry 2022;12:352.
141. Long JM, Maloney B, Rogers JT, Lahiri DK. Novel upregulation of amyloid-β precursor protein (APP) by microRNA-346 via targeting of APP mRNA 5'-untranslated region: Implications in Alzheimer's disease. Mol Psychiatry 2019;24:345-363.
142. Kumar S, Reddy AP, Yin X, Reddy PH. Novel MicroRNA-455-3p and its protective effects against abnormal APP processing and amyloid beta toxicity in Alzheimer's disease. Biochim Biophys Acta Mol Basis D ;1865:2428-40.
143. Hu YB, Zhang YF, Ren RJ, et al. microRNA-425 loss mediates amyloid plaque microenvironment heterogeneity and promotes neurodegenerative pathologies. Aging Cell 2021;20:e13454.
144. Qiu W, Guo X, Lin X, et al. Transcriptome-wide piRNA profiling in human brains of Alzheimer's disease. Neurobiol Aging 2017;57:170-177.
145. Balietti M, Conti F. Environmental enrichment and the aging brain: is it time for standardization? Neurosci Biobehav Rev 2022;139:104728.
146. Liew AKY, Teo CH, Soga T. The molecular effects of environmental enrichment on Alzheimer's disease. Mol Neurobiol 2022;59:7095-118.
147. Griñan-Ferré C, Pérez-Cáceres D, Gutiérrez-Zetina SM, et al. Environmental enrichment improves behavior, cognition, and brain functional markers in young senescence-accelerated prone mice (SAMP8). Mol Neurobiol 2016;53:2435-50.
148. Schmidt S, Haase M, Best L, et al. Restoring age-related cognitive decline through environmental enrichment: a transcriptomic approach. Cells 2022;11:3864.
149. Zhang ZZ, Zeng LP, Chen J, et al. Long-term environmental enrichment relieves dysfunctional cognition and synaptic protein levels induced by prenatal inflammation in older CD-1 mice. Neural Plast 2022;2022:1483101.
150. Herring A, Yasin H, Ambrée O, Sachser N, Paulus W, Keyvani K. Environmental enrichment counteracts Alzheimer's neurovascular dysfunction in TgCRND8 mice. Brain Pathol 2008;18:32-9.
151. Wei Z, Meng X, El Fatimy R, et al. Environmental enrichment prevents Aβ oligomer-induced synaptic dysfunction through mirna-132 and hdac3 signaling pathways. Neurobiol Dis 2020;134:104617.
152. Bourdon E, Belmin J. Enriched gardens improve cognition and independence of nursing home residents with dementia: a pilot controlled trial. Alzheimers Res Ther 2021;13:116.
153. Liśkiewicz A, Przybyła M, Wojakowska A, et al. Physical activity reduces anxiety and regulates brain fatty acid synthesis. Mol Brain 2020;13:62.
154. Tai F, Wang C, Deng X, et al. Treadmill exercise ameliorates chronic REM sleep deprivation-induced anxiety-like behavior and cognitive impairment in C57BL/6J mice. Brain Res Bull 2020;164:198-207.
155. Choi SH, Bylykbashi E, Chatila ZK, et al. Combined adult neurogenesis and BDNF mimic exercise effects on cognition in an Alzheimer's mouse model. Science 2018; 361:eaan8821.
156. Horowitz AM, Fan X, Bieri G, et al. Blood factors transfer beneficial effects of exercise on neurogenesis and cognition to the aged brain. Science 2020;369:167-173.
157. Sakhare A, Stradford J, Ravichandran R, et al. Simultaneous exercise and cognitive training in virtual reality phase 2 pilot study: impact on brain health and cognition in older adults. Brain Plast 2021;7:111-130.
158. Chin LM, Keyser RE, Dsurney J, Chan L. Improved cognitive performance following aerobic exercise training in people with traumatic brain injury. Arch Phys Med Rehabil 2015;96:754-9.
159. Lourenco MV, Frozza RL, de Freitas GB, et al. Exercise-linked FNDC5/irisin rescues synaptic plasticity and memory defects in Alzheimer's models. Nat Med 2019;25:165-75.
160. Freitas GB, Lourenco MV MV, De Felice FG, et al. Protective actions of exercise-related FNDC5/Irisin in memory and Alzheimer's disease. J Neurochem 2020;155:602-11.
161. Azimi M, Gharakhanlou R, Naghdi N, Khodadadi D, Heysieattalab S. Moderate treadmill exercise ameliorates amyloid-β-induced learning and memory impairment, possibly via increasing AMPK activity and up-regulation of the PGC-1α/FNDC5/BDNF pathway. Peptides 2018;102:78-88.
162. Islam MR, Valaris S, Young MF, et al. Exercise hormone irisin is a critical regulator of cognitive function. Nat Metab 2021;3:1058-70.
163. Pignataro P, Dicarlo M, Zerlotin R, et al. FNDC5/Irisin system in neuroinflammation and neurodegenerative diseases: update and novel perspective. Int J Mol Sci 2021;22:1605.
164. Chen K, Wang K, Wang T. Protective effect of irisin against Alzheimer's disease. Front Psychiatry 2022;13:967683.
165. Han R, Liu Z, Sun N, et al. BDNF alleviates neuroinflammation in the hippocampus of type 1 diabetic mice via blocking the aberrant HMGB1/RAGE/NF-κB pathway. Aging Dis 2019;10:611-25.
166. Gao L, Zhang Y, Sterling K, Song W. Brain-derived neurotrophic factor in Alzheimer's disease and its pharmaceutical potential. Transl Neurodegener 2022;11:4.
167. Nigam SM, Xu S, Kritikou JS, Marosi K, Brodin L, Mattson MP. Exercise and BDNF reduce Aβ production by enhancing α-secretase processing of APP. J Neurochem 2017;142:286-96.
168. Noda Y, Kuzuya A, Tanigawa K, et al. Fibronectin type III domain-containing protein 5 interacts with APP and decreases amyloid beta production in Alzheimer’s disease. Mol Brain 2018;11:61.
169. Tan ZX, Dong F, Wu LY, Feng YS, Zhang F. The beneficial role of exercise on treating Alzheimer's disease by inhibiting β-amyloid peptide. Mol Neurobiol 2021;58:5890-06.
170. Brown BM, Peiffer J, Rainey-Smith SR. Exploring the relationship between physical activity, beta-amyloid and tau: a narrative review. Ageing Res Rev 2019;50:9-18.
171. De la Rosa A, Olaso-Gonzalez G, Arc-Chagnaud C, et al. Physical exercise in the prevention and treatment of Alzheimer's disease. J Sport Health Sci 2020;9:394-404.
172. Almeida EJR, Ibrahim HJ, Chitolina Schetinger MR, de Andrade CM, Cardoso AM. Modulation of inflammatory mediators and microglial activation through physical exercise in Alzheimer's and Parkinson's diseases. Neurochem Res 2022;47:3221-40.
173. Ohia-Nwoko O, Montazari S, Lau YS, Eriksen JL. Long-term treadmill exercise attenuates tau pathology in P301S tau transgenic mice. Mol Neurodegener 2014;9:54.
174. Liu HL, Zhao G, Zhang H, Shi LD. Long-term treadmill exercise inhibits the progression of Alzheimer's disease-like neuropathology in the hippocampus of APP/PS1 transgenic mice. Behav Brain Res 2013;256:261-72.
175. Kang EB, Cho JY. Effect of treadmill exercise on PI3K/AKT/mTOR, autophagy, and Tau hyperphosphorylation in the cerebral cortex of NSE/htau23 transgenic mice. J Exerc Nutr Biochem 2015;19:199-209.
176. Peng Y, Chi R, Liu G, Tian W, Zhang J, Zhang R. Aerobic exercise regulates apoptosis through the PI3K/Akt/GSK-3β signaling pathway to improve cognitive impairment in Alzheimer's disease mice. Neural Plast 2022;2022:1500710.
177. Lv J, Jiang S, Yang Z, et al. PGC-1α sparks the fire of neuroprotection against neurodegenerative disorders. Ageing Res Rev 2018;44:8-21.
178. Fernandez-Marcos PJ, Auwerx J. Regulation of PGC-1α, a nodal regulator of mitochondrial biogenesis. Am J Clin Nutr 2011;93:884S-90.
179. Wang R, Li JJ, Diao S, et al. Metabolic stress modulates Alzheimer's β-secretase gene transcription via SIRT1-PPARγ-PGC-1 in neurons. Cell Metab 2013;17:685-94.
180. Katsouri L, Lim YM, Blondrath K, et al. PPARγ-coactivator-1α gene transfer reduces neuronal loss and amyloid-β generation by reducing β-secretase in an Alzheimer's disease model. Proc Natl Acad Sci USA 2016;113:12292-7.
181. Shah SA, Yoon GH, Chung SS, et al. Novel osmotin inhibits SREBP2 via the AdipoR1/AMPK/SIRT1 pathway to improve Alzheimer's disease neuropathological deficits. Mol Psychiatry 2017;22:407-16.
182. Porquet D, Casadesús G, Bayod S, et al. Dietary resveratrol prevents Alzheimer’s markers and increases life span in SAMP8. Age 2013;35:1851-65.
183. Zhang Z, Shen Q, Wu X, Zhang D, Xing D. Activation of PKA/SIRT1 signaling pathway by photobiomodulation therapy reduces Aβ levels in Alzheimer's disease models. Aging Cell 2020;19:e13054.
184. Revilla S, Suñol C, García-Mesa Y, Giménez-Llort L, Sanfeliu C, Cristòfol R. Physical exercise improves synaptic dysfunction and recovers the loss of survival factors in 3xTg-AD mouse brain. Neuropharmacology 2014;81:55-63.
185. Koo JH, Kang EB, Oh YS, Yang DS, Cho JY. Treadmill exercise decreases amyloid-beta burden possibly via activation of SIRT-1 signaling in a mouse model of Alzheimer’s disease. Exp Neurol 2017;288:142-52.
186. Gomez-Pinilla F, Zhuang Y, Feng J, Ying Z, Fan G. Exercise impacts brain-derived neurotrophic factor plasticity by engaging mechanisms of epigenetic regulation. Eur J Neurosci 2011;33:383-90.
187. Intlekofer KA, Berchtold NC, Malvaez M, et al. Exercise and sodium butyrate transform a subthreshold learning event into long-term memory via a brain-derived neurotrophic factor-dependent mechanism. Neuropsychopharmacology 2013;38:2027-34.
188. Sleiman SF, Henry J, Al-Haddad R, et al. Exercise promotes the expression of brain derived neurotrophic factor (BDNF) through the action of the ketone body β-hydroxybutyrate. Elife 2016;5:e15092.
189. Collins A, Hill LE, Chandramohan Y, et al. Exercise improves cognitive responses to psychological stress through enhancement of epigenetic mechanisms and gene expression in the dentate gyrus. PLoS One 2009;4:e4330.
190. Elsner VR, Lovatel GA, Bertoldi K, et al. Effect of different exercise protocols on histone acetyltransferases and histone deacetylases activities in rat hippocampus. Neuroscience 2011;192:580-7.
191. Elsner VR, Lovatel GA, Moysés F, et al. Exercise induces age-dependent changes on epigenetic parameters in rat hippocampus: a preliminary study. Exp Gerontol 2013;48:136-9.
192. Abel JL, Rissman EF. Running-induced epigenetic and gene expression changes in the adolescent brain. Int J Dev Neurosci 2013;31:382-90.
193. Lovatel GA, Elsner VR, Bertoldi K, et al. Treadmill exercise induces age-related changes in aversive memory, neuroinflammatory and epigenetic processes in the rat hippocampus. Neurobiol Learn Mem 2013;101:94-102.
194. Patki G, Solanki N, Atrooz F, et al. Novel mechanistic insights into treadmill exercise based rescue of social defeat-induced anxiety-like behavior and memory impairment in rats. Physiol Behav 2014; 130:135-44.
195. Spindler C, Cechinel LR, Basso C, et al. Treadmill exercise alters histone acetyltransferases and histone deacetylases activities in frontal cortices from wistar rats. Cell Mol Neurobiol 2014;34:1097-101.
196. Cosín-Tomás M, Alvarez-López MJ, Sanchez-Roige S, et al. Epigenetic alterations in hippocampus of SAMP8 senescent mice and modulation by voluntary physical exercise. Front Aging Neurosci 2014;6:51.
197. Bao TH, Miao W, Han JH, et al. Spontaneous running wheel improves cognitive functions of mouse associated with miRNA expressional alteration in hippocampus following traumatic brain injury. J Mol Neurosci 2014;54:622-9.
198. Hu T, Zhou FJ, Chang YF, et al. miR21 is Associated with the Cognitive improvement following voluntary running wheel exercise in TBI mice. J Mol Neurosci 2015;57:114-22.
199. Pan-Vazquez A, Rye N, Ameri M, et al. Impact of voluntary exercise and housing conditions on hippocampal glucocorticoid receptor, miR-124 and anxiety. Mol Brain 2015;8:40.
200. Ieraci A, Mallei A, Musazzi L, Popoli M. Physical exercise and acute restraint stress differentially modulate hippocampal brain-derived neurotrophic factor transcripts and epigenetic mechanisms in mice. Hippocampus 2015;25:1380-92.
201. Zhong T, Ren F, Huang CS, et al. Swimming exercise ameliorates neurocognitive impairment induced by neonatal exposure to isoflurane and enhances hippocampal histone acetylation in mice. Neuroscience 2016;316:378-88.
202. Kim TK, Lee JE, Kim JE, et al. G9a-Mediated regulation of OXT and AVP expression in the basolateral amygdala mediates stress-induced lasting behavioral depression and its reversal by exercise. Mol Neurobiol 2016;53:2843-56.
203. Maejima H, Kanemura N, Kokubun T, Murata K, Takayanagi K. Exercise enhances cognitive function and neurotrophin expression in the hippocampus accompanied by changes in epigenetic programming in senescence-accelerated mice. Neurosci Lett 2018;665:67-73.
204. Sølvsten CAE, de Paoli F, Christensen JH, Nielsen AL. Voluntary physical exercise induces expression and epigenetic remodeling of vegfa in the rat hippocampus. Mol Neurobiol 2018;55:567-82.
205. Li X, Inoue T, Hayashi M, Maejima H. Exercise enhances the expression of brain-derived neurotrophic factor in the hippocampus accompanied by epigenetic alterations in senescence-accelerated mice prone 8. Neurosci Lett 2019;706:176-81.
206. de Meireles LCF, Galvão F Jr, Walker DM, et al. Exercise modalities improve aversive memory and survival rate in aged rats: role of hippocampal epigenetic modifications. Mol Neurobiol 2019;56:8408-19.
207. Zhang J, Li J, Zhu Y, Miao Z, Tian Y. Forced running exercise mitigates radiation-induced cognitive deficits via regulated DNA hydroxymethylation. Epigenomics 2020;12:385-96.
208. Davaa G, Hong JY, Kim TU, Lee SJ, Kim SY, Hong K, Hyun JK. Exercise ameliorates spinal cord injury by changing DNA methylation. Cells 2021;10:143.
209. Maejima H, Kitahara M, Takamatsu Y, Mani H, Inoue T. Effects of exercise and pharmacological inhibition of histone deacetylases (HDACs) on epigenetic regulations and gene expressions crucial for neuronal plasticity in the motor cortex. Brain Res 2021;1751:147191.
210. Liu SJ, Cai TH, Fang CL, et al. Long-term exercise training down-regulates m6A RNA demethylase FTO expression in the hippocampus and hypothalamus: an effective intervention for epigenetic modification. BMC Neurosci 2022;23:54.
211. Barrès R, Yan J, Egan B, et al. Acute exercise remodels promoter methylation in human skeletal muscle. Cell Metab 2012;15:405-11.
212. Bajpeyi S, Covington JD, Taylor EM, Stewart LK, Galgani JE, Henagan TM. Skeletal Muscle PGC1α -1 Nucleosome Position and -260 nt DNA Methylation Determine Exercise Response and Prevent Ectopic Lipid Accumulation in Men. Endocrinology 2017;158:2190-9.
213. Yu M, Stepto NK, Chibalin AV, et al. Metabolic and mitogenic signal transduction in human skeletal muscle after intense cycling exercise. J Physiol 2003;546:327-35.
214. Smith JA, Kohn TA, Chetty AK, Ojuka EO. CaMK activation during exercise is required for histone hyperacetylation and MEF2A binding at the MEF2 site on the Glut4 gene. Am J Physiol Endocrinol Metab 2008;295:E698-704.
215. Chandramohan Y, Droste SK, Arthur JS, Reul JM. The forced swimming-induced behavioural immobility response involves histone H3 phospho-acetylation and c-Fos induction in dentate gyrus granule neurons via activation of the N-methyl-D-aspartate/extracellular signal-regulated kinase/mitogen- and stress-activated kinase signalling pathway. Eur J Neurosci 2008;27:2701-13.
216. Gomez-Pinilla F, Zhuang Y, Feng J, Ying Z, Fan G. Exercise impacts brain-derived neurotrophic factor plasticity by engaging mechanisms of epigenetic regulation. Eur J Neurosci 2011;33:383-90.
217. Mackay CP, Kuys SS, Brauer SG. The effect of aerobic exercise on brain-derived neurotrophic factor in people with neurological disorders: a systematic review and meta-analysis. Neural Plast 2017;2017:4716197.
218. Wang R, Holsinger RMD. Exercise-induced brain-derived neurotrophic factor expression: Therapeutic implications for Alzheimer's dementia. Ageing Res Rev 2018; 48:109-121.
219. Erickson KI, Voss MW, Prakash RS, et al. Exercise training increases size of hippocampus and improves memory. Proc Natl Acad Sci USA 2011;108:3017-22.
220. Maass A, Düzel S, Brigadski T, et al. Relationships of peripheral IGF-1, VEGF and BDNF levels to exercise-related changes in memory, hippocampal perfusion and volumes in older adults. Neuroimage 2016;131:142-54.
221. Wrann CD, White JP, Salogiannnis J, et al. Exercise induces hippocampal BDNF through a PGC-1α/FNDC5 pathway. Cell Metab 2013;18:649-59.
222. El Hayek L, Khalifeh M, Zibara V, et al. Lactate mediates the effects of exercise on learning and memory through sirt1-dependent activation of hippocampal brain-derived neurotrophic factor (BDNF). J Neurosci 2019;39:2369-82.
223. Fakhraei S, Reza Almasi M, Peeri M, Gharakhanlou R. The effect of 4-week rehabilitation by aerobic exercise on hippocampus BDNF and TGF-β1 gene expressions inAβ 1-42-induced rat model of Alzheimer's disease. J Clin Neurosci 2022;95:106-11.
224. Nigam SM, Xu S, Kritikou JS, Marosi K, Brodin L, Mattson MP. Exercise and BDNF reduce Aβ production by enhancing α-secretase processing of APP. J Neurochem 2017;142:286-96.
225. Baranowski BJ, Hayward GC, Marko DM, MacPherson REK. Examination of BDNF treatment on BACE1 activity and acute exercise on brain BDNF signaling. Front Cell Neurosci 2021;15:665867.
226. Nott A, Watson PM, Robinson JD, Crepaldi L, Riccio A. S-Nitrosylation of histone deacetylase 2 induces chromatin remodelling in neurons. Nature 2008;455:411- 5.
227. Vaynman S, Ying Z, Gomez-Pinilla F. Interplay between brain-derived neurotrophic factor and signal transduction modulators in the regulation of the effects of exercise on synaptic-plasticity. Neuroscience 2003;122:647-57.
228. Vaynman S, Ying Z, Gomez-Pinilla F. The select action of hippocampal calcium calmodulin protein kinase II in mediating exercise-enhanced cognitive function. Neuroscience 2007;144:825-33.
229. Zocchi L, Sassone-Corsi P. SIRT1-mediated deacetylation of MeCP2 contributes to BDNF expression. Epigenetics 2012;7:695-700.
230. Marton O, Koltai E, Takeda M, et al. The rate of training response to aerobic exercise affects brain function of rats. Neurochem Int 2016;99:16-23.
231. Steiner JL, Murphy EA, McClellan JL, Carmichael MD, Davis JM. Exercise training increases mitochondrial biogenesis in the brain. J Appl Physiol 2011;111:1066-71.
232. Lee HR, Shin HK, Park SY, et al. Cilostazol suppresses β-amyloid production by activating a disintegrin and metalloproteinase 10 via the upregulation of SIRT1-coupled retinoic acid receptor-β. J Neurosci Res 2014;92:1581-90.
233. Min SW, Sohn PD, Li Y, et al. SIRT1 deacetylates Tau and reduces pathogenic Tau spread in a mouse model of tauopathy. J Neurosci 2018;38:3680-8.
234. Dungan CM, Valentino T, Vechetti IJ Jr, et al. Exercise-mediated alteration of hippocampal Dicer mRNA and miRNAs is associated with lower BACE1 gene expression and Aβ(1-42) in female 3xTg-AD mice. J Neurophysiol 2020;124:1571-7.
235. Wang X, Liu P, Zhu H, et al. miR-34a, a microRNA up-regulated in a double transgenic mouse model of Alzheimer's disease, inhibits bcl2 translation. Brain Res Bull 2009;80:268-73.
236. Jian C, Lu M, Zhang Z, et al. miR-34a knockout attenuates cognitive deficits in APP/PS1 mice through inhibition of the amyloidogenic processing of APP. Life Sci 2017;182:104-11.
237. Sarkar S, Engler-Chiurazzi EB, Cavendish JZ, et al. Over-expression of miR-34a induces rapid cognitive impairment and Alzheimer's disease-like pathology. Brain Res 2019;1721:146327.
238. Xu L, Zheng YL, Yin X, et al. Excessive treadmill training enhances brain-specific microrna-34a in the mouse hippocampus. Front Mol Neurosci 2020;13:7.
239. Kou X, Li J, Liu X, et al. Swimming attenuates d-galactose-induced brain aging via suppressing miR-34a-mediated autophagy impairment and abnormal mitochondrial dynamics. J Appl Physiol 2017;122:1462-9.
240. Lin Y, Shen J, Li D, et al. MiR-34a contributes to diabetes-related cochlear hair cell apoptosis via SIRT1/HIF-1α signaling. Gen Comp Endocrinol 2017;246:63-70.
241. Zhang H, Zhang XM, Zong DD, et al. miR-34a-5p up-regulates the IL-1β/COX2/PGE2 inflammation pathway and induces the release of CGRP via inhibition of SIRT1 in rat trigeminal ganglion neurons. FEBS Open Bio 2021;11:300-11.
242. Wang RY, Phang RZ, Hsu PH, Wang WH, Huang HT, Liu IY. In vivo knockdown of hippocampal miR-132 expression impairs memory acquisition of trace fear conditioning. Hippocampus 2013;23:625-33.
243. Scott HL, Tamagnini F, Narduzzo KE, et al. MicroRNA-132 regulates recognition memory and synaptic plasticity in the perirhinal cortex. Eur J Neurosci 2012;36:2941-8.
244. Hansen KF, Karelina K, Sakamoto K, Wayman GA, Impey S, Obrietan K. miRNA-132: a dynamic regulator of cognitive capacity. Brain Struct Funct 2013;218:817-31.
245. Radom-Aizik S, Zaldivar F, Leu SY, Adams GR, Oliver S, Cooper DM. Effects of exercise on microRNA expression in young males peripheral blood mononuclear cells. Clin Transl Sci 2012;5:32-8.
246. Dong J, Liu Y, Zhan Z, Wang X. MicroRNA-132 is associated with the cognition improvement following voluntary exercise in SAMP8 mice. Brain Res Bull 2018;140:80-7.
247. Sadeghian R, Shahidi S, Komaki A, et al. Synergism effect of swimming exercise and genistein on the inflammation, oxidative stress, and VEGF expression in the retina of diabetic-ovariectomized rats. Life Sci 2021;284:119931.
248. Shi L, Zhang R, Li T, et al. Decreased miR-132 plays a crucial role in diabetic encephalopathy by regulating the GSK-3β/Tau pathway. Aging 2020;13:4590-604.
249. Tan Z, Chen Y, Xie W, Liu X, Zhu Y, Zhu Y. Nimodipine attenuates tau phosphorylation at Ser396 via miR-132/GSK-3β pathway in chronic cerebral hypoperfusion rats. Eur J Pharmacol 2018;819:1-8.
250. Qu J, Xiong X, Hujie G, Ren J, Yan L, Ma L. MicroRNA-132-3p alleviates neuron apoptosis and impairments of learning and memory abilities in Alzheimer's disease by downregulation of HNRNPU stabilized BACE1. Cell Cycle 2021;20:2309-2320.
251. Smith PY, Hernandez-Rapp J, Jolivette F, et al. miR-132/212 deficiency impairs tau metabolism and promotes pathological aggregation in vivo. Hum Mol Genet 2015;24:6721-35.
252. Hernandez-Rapp J, Rainone S, Goupil C, et al. microRNA-132/212 deficiency enhances Aβ production and senile plaque deposition in Alzheimer's disease triple transgenic mice. Sci Rep 2016;6:30953.
253. Nielsen S, Åkerström T, Rinnov A, et al. The miRNA plasma signature in response to acute aerobic exercise and endurance training. PLoS One 2014;9:e87308.
254. Sierksma A, Lu A, Salta E, et al. Deregulation of neuronal miRNAs induced by amyloid-β or TAU pathology. Mol Neurodegener 2018;13:54.
255. Su W, Aloi MS, Garden GA. MicroRNAs mediating CNS inflammation:small regulators with powerful potential. Brain Behav Immun 2016;52:1-8.
256. Liang C, Zou T, Zhang M, et al. MicroRNA-146a switches microglial phenotypes to resist the pathological processes and cognitive degradation of Alzheimer's disease. Theranostics 2021;11:4103-21.
257. Maffioletti E, Milanesi E, Ansari A, et al. miR-146a plasma levels are not altered in Alzheimer's disease but correlate with age and illness severity. Front Aging Neurosci 2020;11:366.
258. Ansari A, Maffioletti E, Milanesi E, et al. miR-146a and miR-181a are involved in the progression of mild cognitive impairment to Alzheimer's disease. Neurobiol Aging 2019;82:102-9.
259. Olcum M, Tufekci KU, Durur DY, et al. Ethyl pyruvate attenuates microglial NLRP3 inflammasome activation via inhibition of HMGB1/NF-κB/miR-223 signaling. Antioxidants 2021;10:745.
260. La Rosa F, Mancuso R, Agostini S, et al. Pharmacological and epigenetic regulators of NLRP3 inflammasome activation in Alzheimer's disease. Pharmaceuticals 2021;14:1187.
261. Jia LH, Liu YN. Downregulated serum miR-223 servers as biomarker in Alzheimer's disease. Cell Biochem Funct 2016;34:233-7.
262. Wei H, Xu Y, Chen Q, Chen H, Zhu X, Li Y. Mesenchymal stem cell-derived exosomal miR-223 regulates neuronal cell apoptosis. Cell Death Dis 2020;11:290.
263. Harraz MM, Eacker SM, Wang X, Dawson TM, Dawson VL. MicroRNA-223 is neuroprotective by targeting glutamate receptors. Proc Natl Acad Sci USA 2012; 109:18962-7.
Cite This Article
Export citation file: BibTeX | RIS
OAE Style
Li S. Benefits of physical exercise on Alzheimer's disease: an epigenetic view. Ageing Neur Dis 2023;3:6. http://dx.doi.org/10.20517/and.2022.37
AMA Style
Li S. Benefits of physical exercise on Alzheimer's disease: an epigenetic view. Ageing and Neurodegenerative Diseases. 2023; 3(2): 6. http://dx.doi.org/10.20517/and.2022.37
Chicago/Turabian Style
Li, Song. 2023. "Benefits of physical exercise on Alzheimer's disease: an epigenetic view" Ageing and Neurodegenerative Diseases. 3, no.2: 6. http://dx.doi.org/10.20517/and.2022.37
ACS Style
Li, S. Benefits of physical exercise on Alzheimer's disease: an epigenetic view. Ageing. Neur. Dis. 2023, 3, 6. http://dx.doi.org/10.20517/and.2022.37
About This Article
Copyright
Data & Comments
Data
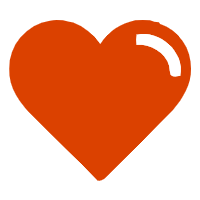

Comments
Comments must be written in English. Spam, offensive content, impersonation, and private information will not be permitted. If any comment is reported and identified as inappropriate content by OAE staff, the comment will be removed without notice. If you have any queries or need any help, please contact us at support@oaepublish.com.