Scaffold tissue engineering strategies for volumetric muscle loss
Abstract
Volumetric muscle loss (VML) refers to a composite, en bloc loss of skeletal muscle mass resulting in functional impairment. These injuries normally heal with excessive fibrosis, minimal skeletal muscle regeneration, and poor functional recovery. Functional muscle transfer is a treatment option for some patients but is limited both by the degree of functional restoration as well as donor site morbidity. As such, new therapeutic options are necessary. De novo regeneration of skeletal muscle, by way of tissue engineering, is an emerging strategy to treat VML. This review evaluates available scaffolds for promoting skeletal muscle regeneration and functional recovery following VML. The use of growth factors and stem cell therapies, which may augment scaffold integration and skeletal muscle reconstitution, are also discussed. Regenerative medicine with the use of scaffolds is a promising area in skeletal muscle reconstruction and VML treatment.
Keywords
INTRODUCTION
Volumetric muscle loss (VML) following trauma or surgical resection is characterized by irreversible damage or loss of composite skeletal muscle tissue[1,2]. VML injuries can be particularly morbid when involving the lower extremities, as they significantly impair ambulation[3,4]. Critical sized loss of skeletal muscle tissue (20% of muscle volume), as seen in substantial VML injuries, overwhelms the natural reparative and regenerative ability within skeletal muscle[3,5,6]. Instead of muscle regeneration, VML injuries normally heal with substantial fibrosis, permanent muscle damage, and poor extremity function. These sequelae significantly detract from the patient’s ability to perform daily activities, ambulate, and reestablish quality of life[1,2,7-9]. An example of a patient with VML injury from a pre-tibial sarcoma is described [Figure 1].
Figure 1. VML in Patient. (A) Patient presented with sarcoma in pre-tibial region; (B) Tumor extirpation results in loss of medial soleus, gastrocnemius, and tibialis anterior muscle; (C) Reconstruction utilizing non-functioning free muscle transfer. Patient exhibits permanent weakness in foot extension and flexion.
Treatment options following extremity VML injury remain limited[2,10]. The most common treatment to restore strength across an injured muscle is free or pedicled functional muscle transfer. However, this results in incomplete functional recovery and involves donor site morbidity and weakness[11-17]. Targeted physical therapy promotes muscle regeneration and healing following VML, but only results in partial recovery of the original function[10,18]. Novel tissue engineering strategies, in place of autologous muscle transfer, are key to skeletal muscle regeneration and functional recovery following VML injuries[19-22]. This review will evaluate current tissue engineering strategies using scaffolds to promote skeletal muscle recovery in the treatment of VML.
PATHOPHYSIOLOGY OF VML
VML results in limited muscle fiber regeneration, substantial functional limitation and disability, and excessive fibrosis[1,4,23]. Muscle regeneration in VML pathology is insufficient due to the loss of essential regenerative elements such as growth factors, intact basal lamina of the extracellular matrix (ECM), and stem cells[1]. Skeletal muscle stem cells (MuSCs) are required for skeletal muscle regeneration and are activated by signals from growth factors to enter the cell cycle and proliferate in response to injury[24,25]. Broadly, skeletal muscle regeneration is initiated with pro-inflammatory M1 macrophages that phagocytose necrotic myofibers and activate quiescent MuSCs[26,27]. Anti-inflammatory M2 macrophages then replace M1 macrophages over the next week and promote tissue regeneration by supporting myoblast proliferation, growth, and differentiation[26,27]. The significant loss of MuSCs and a disrupted basal lamina in VML pathology overwhelm skeletal muscles’ innate repair mechanism and result in a paucity of skeletal muscle regeneration following VML injury[4,19,22,28]. Additionally, growth factors, such as insulin growth factor 1 (IGF-1), hepatocyte growth factor (HGF), and fibroblast growth factor 2 (FGF-2), that normally activate MuSCs to enter the cell cycle and proliferate, are downregulated[28-38]. Macrophage-mediated secretion of inflammatory cytokines, such as tumor necrosis factor-alpha (TNF-α) and interleukin 6 (IL-6), which increase myoblast proliferation and differentiation and promote muscle regeneration, is also lacking in VML pathology[24,35,39-44]. Taken together, the cells and growth factors required for myogenesis are deficient in skeletal muscle following VML, severely impairing functional reconstitution of the muscle.
In contrast, fibrogenic cytokines, such as transforming growth factor β1 (TGF-β1), are upregulated and result in a pathologic proliferation of fibroblasts and ECM components (collagen, laminin, and fibronectin), leading to extensive fibrosis[3,23,35,44]. Fibrosis further prevents normal neuron and vasculature ingrowth, resulting in denervated and ischemic muscle with little elasticity, loss of strength, and impaired contraction and relaxation[5,40]. As such, VML injuries exhibit minimal restoration of strength as myogenesis is diminished. Fibrosis further limits muscle strength and excursion, reinnervation, and revascularization within the site of VML injury.
TISSUE-ENGINEERED SCAFFOLDS FOR SKELETAL MUSCLE REGENERATION
Tissue engineering combines scaffolds, cells, and biochemical cues to aid in tissue regeneration and repair as a treatment for VML. Scaffolds are three-dimensional (3D) structural constructs that support ECM deposition, limit fibrosis, promote skeletal muscle regeneration, and augment functional muscle recovery[11,18,19,45,46]. Broadly, scaffolds serve as a template for tissue formation and are composed of synthetic or natural biological materials[45]. We will review tissue engineering approaches with hydrogel, acellular, nanofibrous, and electroconductive scaffolds.
The two main approaches to using scaffolds to treat VML are (1) in vivo skeletal muscle regeneration using scaffold development and (2) implantation of an ex vivo skeletal muscle construct [Figure 2][47,48]. Firstly, in vivo tissue engineering involves seeding of host-derived progenitor cells into the scaffold and then transplantation into the defect. Low viability, retention, and immune rejection of the seeded cells are some limitations of this technique[47,49]. For ex vivo tissue engineering, a functionally mature construct of contractile myofibers developed from ex vivo culture of scaffolds, biological factors, and progenitor cells is implanted into the muscle defect[50]. However, the contractile force produced by scaffolds and oxygen and nutrient diffusion to support cell viability is significantly lower than that of native muscle tissue[51,52]. Addressing these limitations is necessary to make scaffold therapy a reliable option for VML treatment.
Figure 2. Tissue engineering approaches in treatment of volumetric muscle loss. In an in vivo approach, progenitor cells are obtained from the host to be seeded into the scaffolds, together with incorporation of biochemical cues (such as growth factors), the construct is immediately applied to the defect. In ex vivo tissue engineering, the same materials are first incubated together in a bioreactor so that a differentiated and functional construct is produced prior to implantation. Figure was created with Google Drawing.
Scaffold design and considerations
Scaffolds are constructed extracellular matrices that help direct muscle regeneration and optimize functional recovery [Figure 3]. The choice of material, composition of scaffolds, growth factor integration, and cellularity can all be modified in scaffold-based VML treatment[25,27]. Changes in each of these properties provide both advantages and limitations. Scaffold architecture directly modulates cell adhesion, morphology, orientation, migration, proliferation, genetic expression, and differentiation[53,54]. The porosity of scaffolds modulates nutrient exchange and oxygen transport and facilitates cell seeding, penetration, and distribution[55-58]. Balanced rates of scaffold degradation to tissue growth maintain structural stability with increasing mechanical stress until the tissue can maintain its structure without additional support[27,59]. Biologically active molecules such as growth factors and cytokines can further regulate muSC function and behavior[39,40]. Engineered scaffolds can also be utilized to deliver MuSCs and initiate direct tissue repair and regeneration in the area of injury[25,60,61].
Figure 3. Scaffold implantation into VML injury in a rat. (A) VML injury to rat tibialis anterior using a 6 mm punch biopsy; (B) Acellular collagen glycosaminoglycan scaffold implantation to the defect[62].
Scaffolds as a mechanism for cell and growth factor delivery
While acellular scaffolds alone have demonstrated improvement in endogenous skeletal muscle regeneration through recruitment and proliferation of host cell populations, they result in incomplete functional recovery with sub-optimal muscle tissue regeneration and scaffold integration[60,63]. To improve muscle regeneration, scaffolds can be used to deliver growth factors to promote myogenesis following VML[25,27,64]. IGF-1 and basic fibroblast growth factor (bFGF) have previously been shown to improve healing following muscle injury[40,65]. In the context of VML pathology, TNF-α and IL-6, and growth factors IGF-1, HGF, vascular endothelial growth factor (VEGF), and FGF-2 have been studied for skeletal muscle regeneration following VML injury[25,66-75]. Controlled release of growth factors through scaffold materials over time is more effective than single bolus dosing and results in improved muscle regeneration[76,77]. Similarly, intramuscular injection of cells allows for local engraftment and prevents widespread distribution of cells, but local engraftment[78]. Progenitor cell populations other than MuSCs have also been studied for the reconstruction of VML, including myoblasts[79-81].
In preclinical studies, hydrogels in conjunction with IGF-1 and bFGF showed significant improvements in muscle formation and functional recovery in a murine latissimus dorsi VML model compared to hydrogels alone, hydrogels with MPCs, and keratin hydrogels with MPCs, bFGF, and IGF-1[80]. A correct ratio of cells and growth factors remains unclear. Perivascular stem cells (PSCs) and mesenchymal stem cells (MSCs) have also demonstrated improved myogenesis in the area of VML injury, and a fibrin-laminin hydrogel with MSCs improved muscle mass and myogenic marker expression[82-85]. Scaffold delivery of combinations of growth factors and progenitor cells is a promising option for VML therapy[25,77,86].
HYDROGEL SCAFFOLDS
Hydrogels are 3D networks of hydrophilic synthetic or natural polymer chains. They are a popular choice of scaffold due to their easily manipulated physical and chemical properties that mimic the native ECM[87-89]. ECM-derived biomaterials commonly used to create hydrogel scaffolds include collagen, fibrin, keratin, polysaccharides, and alginate, but hydrogels can be synthetic or a combination of both to allow for more durability and mechanical strength[55,90-92]. An acellular hydrogel containing methacrylic acid significantly increased muscle fiber growth with a significant 1.5-fold increase in torque production, vascularization, and innervation in murine tibialis anterior (TA) VML injury model (P < 0.01)[93]. In vivo incorporation of growth factors and progenitor cells in hydrogels into the targeted area of injury can also be used to promote cell viability, myogenic differentiation, and angiogenesis. One study involving keratin hydrogels with IGF-1 and bFGF demonstrated significantly greater recovery contractile force than in keratin hydrogels with MPCs, with about 70% of native muscle force, in a murine latissimus dorsi model of VML injury[80]. Myoblasts with IGF-1, FGF, and VEGF delivered in vivo using keratose/alginate hydrogels and myoblasts seeded into fibrin hydrogels alone both demonstrated myogenesis, reduced scar tissue, and construct vascularization in animal models on VML[81,94-97]. Delivery of MuSCs using a polymer scaffold causes significantly higher engraftment of cells into host muscle compared to direct injection into the defect[61,98]. Muscle-derived stem cells seeded onto collagen scaffolds showed a significant 1.5-fold increase in cross-sectional area of rectus femoris muscle at 8 weeks post-injury compared to untreated VML in a murine model of VML[99]. Mesenchymal stem cells in a fibrin-laminin scaffold demonstrated an 8.2% increase in normalized muscle mass and significantly increased myofibers compared to the untreated group in a gastrocnemius-soleus murine model of VML[85]. Manipulation of biomaterials in hydrogels to influence cell behavior, improve mechanical strength, and reduce host immune response is a key area of interest. Combinations of synthetic, such as polycaprolactone, and natural materials are researched to enhance hydrogels’ mechanical strength and increase myogenesis in VML models[87,91,92,100]. Adjusting crosslinking modulates hydrogel strength; chemical crosslinking reinforces mechanical strength in contrast to physical crosslinking[57,101,102]. The components of hydrogels such as collagen, gelatin, and alginate or polyethylene glycol correspond to fibrous, microporous, and nanoporous architectures, which subsequently influence cellular migration, proliferation, and nutrient exchange[57,58,103]. Biomaterials can be modified with immunomodulatory genes and the selection of biomaterial based on patient age and sexuality are two studies of interest[104,105]. Induced pluripotent stem cells (iPSCs), adult somatic cells that has been reprogrammed to become pluripotent, have gained traction due to their immunocompatibility and differentiation potential, and delivery of iPSCs using fibrin hydrogels have demonstrated improved in situ muscle contractility and improved engraftment of host myofibers and MuSCs in a VML murine model[106]. Hydrogels are very tunable, but the ideal biomaterial and combination of progenitor stem cells and growth factors for large-scale muscle regeneration have yet to be achieved.
DECELLULARIZED SCAFFOLDS
Decellularized scaffolds are comprised of native ECM components after the removal of all tissue cellular components[107-109] [Table 1]. As such, these scaffolds precisely mimic native tissue architecture. Skeletal muscle ECM is key to constructive remodeling in muscle regeneration as it influences cellular adhesion, signaling, and proliferation and is a major source of growth factors to recreate the complex architecture of muscle tissue[109,110]. Acellular scaffolds utilizing porcine urinary bladder ECM have demonstrated improved migration of PSCs to the injury site with de novo skeletal muscle cell formation and functional improvement in both a murine model for VML and three out of five patients with extremity VML injuries[108,111]. In another small-scale clinical study of 13 patients with injuries to a variety of muscles, implantation of three porcine-derived acellular scaffolds demonstrated an average improvement of 37.3% in strength, a 27.1% enhancement in functional range-of-motion tasks, and a 27.2% increase in bulk muscle at six months[112]. However, conflicting results exist regarding the use of decellularized scaffolds for the treatment of VML[11,45,63]. Porcine urinary bladder ECM in a rat TA VML injury model showed 100-fold less myosin-positive fibers compared to those in the autograft at two, eight, and sixteen weeks post-injury, indicating insufficient muscle fiber regeneration[110]. When normalized to uninjured contralateral muscles, functional recovery, defined by the maximal isometric torque of TA, in the acellular scaffold was significantly less than that of the autograft[110]. Similarly, decellularized scaffolds implanted into a rat TA VML injury model did not show de novo muscle regeneration, characterized by myosin-positive fibers, but instead had increased fibrotic tissue in the injury site at eight weeks post-injury compared to minced muscle scaffolds that showed substantial muscle regeneration[113]. Maximal tetanic isometric TA muscle torque was assessed in vivo, and similarly showed 15% more torque production with the minced muscle scaffolds compared to that of decellularized scaffolds[113].
Comparison of techniques to treat VML
Technique | Advantages | Disadvantages | Takeaways |
Free muscle transfer | -Some functional restoration and volume recovery -Currently used in clinics[13,23] | -Donor site morbidity -Incomplete functional recovery -Lack of donor tissue -Requires highly skilled surgical team[11-17] | -Current standard of treatment for VML -Other techniques are necessary to achieve better function and muscle volume |
Acellular scaffolds | -Native ECM is retained -Augments natural recruitment of progenitor cells -Minimizes host immunogenicity -Tissue-specific ECM, has been used in small-scale clinical studies[109,111,112] | -Decellularization process must be thorough to avoid an adverse host immune response -Ability to regenerate sufficient muscle volume and restoration of function is still incomplete[27,60] | -Improvements in functionality and muscle regeneration in few clinical studies -Fast to produce and shelf-ready -May have the quickest path for approval for commercialization[112] |
Cellular scaffolds | -Increased delivery of progenitor cells supports recovery and regeneration -Easily manipulated architecture and biomaterials[27,61] | Has-only been studied in in vitro and in vivo animal models, -Ability to regenerate sufficient muscle volume and restoration of function is still incomplete[27,123] | -Most promising avenue for skeletal muscle tissue engineering -Various combinations of cells, growth factors, and biomaterials can be incorporated |
Collectively, decellularized or acellular scaffolds may be unable to regenerate sufficient muscle tissue for VML therapy[60,110]. One study on a rat model of VML compared acellular muscle ECM and minced muscle grafts and found no appreciable muscle regeneration, increased collagen deposition/fibrosis, and reduced muSC presence in rats with acellular muscle ECM at 8 weeks[113]. Incorporation of progenitor cells into acellular scaffolds has been proposed as a solution. Progenitor cell delivery using acellular ECM, including MSCs, myoblasts, and MuSCs, has demonstrated improved skeletal muscle regeneration, functional recovery, and angiogenesis at the injury site in animal models[114-119]. The addition of murine myoblasts in a murine TA VML injury model showed a significant increase in muscle volume, mass, and myofiber density compared to scaffolds without the incorporation of myoblasts.[120] Previous rodent studies have indicated that the reduced density of MuSCs in the ECM scaffold may have contributed to the limited muscle regeneration seen after scaffold implantation[60,110,113]. Recent strategies maximize muscle growth and vessel/nerve vascularization. One study aimed to reduce scar tissue and demonstrated reduced fibrosis and improved myofiber regeneration using a decellularized muscle aponeurosis scaffold that distributed mechanical stiffness[121]. An acellular laminin-enriched fibrin scaffold demonstrated improved myofiber regeneration and an average 60% increase in torque production in a rat TA model of VML[122]. More studies are needed to determine the effectiveness of acellular and decellularized scaffolds for muscle regeneration and functional recovery.
NANOFIBROUS SCAFFOLDS
Nanofibrous scaffolds expand tissue engineering capabilities to emulate the ECM architecture at the nanometer scale and guide cell adhesion and proliferation[124]. Nanofibrous constructs, made of synthetic or natural biomaterials, have a desirable high surface area to volume ratio and high porosity and are created through electrospinning, self-assembly, or phase separation[124,125]. Nanofibers, through electrospinning, produce an anisotropic microenvironment that guides geometric myoblast alignment to favor myoblast fusion and muscle regeneration[126,127]. Electrospinning produces anisotropic, geometrically aligned nanofibers that mimic native ECM morphology and function[127,128]. Electrospun nanofiber orientation can guide MSC and fibroblast cell growth and may be preferred in tissue-engineered scaffolds[127,128]. Very recently, one study developed an injectable, anisotropic, nanofibrous hydrogel with magnetic controlled short nanofibers to guide cell alignment and organization using a remote magnetic field. These anisotropic scaffolds significantly improved the alignment of myofibers in vivo and functional recovery in a rat TA VML model[129].
Vascular and nerve regeneration have been explored with nanofibrous scaffolds. One study involving spatially patterned aligned myotubes from an in vitro co-culture of murine myoblasts and vascular endothelial cells in nanofibrillar scaffolds[130]. Implantation of the organized skeletal muscle into a mouse TA VML injury model resulted in highly organized myofibers and increased vascularization and synchronized contractility compared to endothelialized muscle tissue from non-aligned scaffolds, highlighting the potential for improvements in angiogenesis in scaffold tissue engineering[130]. Another study over core-shell composite scaffolds, with a nanofiber yarn core and hydrogel shell that are seeded with myoblasts, and demonstrated both enhanced myofiber alignment and elongation[131]. Pre-innervated scaffolds using co-cultured spinal motor neurons and myocytes in aligned nanofibrous scaffolds in a rat VML model showed greatly increased MuSCs, myocyte fusion and mature neuromuscular junction (NMJs), and muscle regeneration, indicating great potential for pre-innervated scaffolds to treat VML[21]. Cell infiltration is a key limitation in electrospun scaffolds, but adjusting biomaterial selection and variations in electrospinning and post-processing procedures are used to account for this drawback[125,132-136]. Further research is necessary to explore engineered nanofibrous scaffolds to improve spatial organization, vascularization, and innervation of regenerated muscle tissue [Table 2].
Comparison of different types of scaffolds
Scaffold type | Advantages | Disadvantages |
Hydrogel | -Easily manipulated physical and chemical properties that mimic the native ECM[87-89] -Composed of a variety of synthetic and natural materials (collagen, gelatin, fibrin, etc.) that influence cellular migration, proliferation, and nutrient exchange[57,58,103] | -Highly tunable nature of hydrogels leads to wide variability of results between studies -Limited vascularization and innervation capability compared to nanofibrous scaffolds |
Nanofibrous | -Emulate the ECM architecture at the nanometer scale[124,125] -Guide cell adhesion and proliferation at the nanometer scale -High porosity and surface area: volume ratio -Improved myofiber alignment[126,127] -Capability for pre-innervated scaffolds[130] | -Electrospun scaffolds have poor cell infiltration and migration[125,132-136] -Low mechanical strength of scaffold -Less tunable than hydrogels |
Electroconductive | -Enhances regeneration of aligned myofibers, leading to contractile function recovery[12,138-140] -Support contractile force and myoblast differentiation[141-143] | -Carbon-containing scaffolds possess potential cytotoxicity[148-150] -Less tunable than hydrogels |
ELECTROCONDUCTIVE SCAFFOLDS
Electroconductive scaffolds incorporate conductive materials such as carbon nanotubes, graphene, and conductive nanopolymers to mimic the electrical properties of native ECM[137]. The addition of electrical properties to scaffolds enhances the regeneration of aligned myofibers, leading to contractile function recovery, which is currently missing in natural and synthetic biomaterial-based scaffolds[12,138-140]. Electrically stimulated in vitro skeletal muscle constructs improved contractile force, supported myoblast differentiation into myotubes, and increased the size of myobundles[141-143]. Graphene hydrogels have become increasingly popular and have been shown to improve myoblast and fibroblast proliferation and differentiation in vitro[144-146]. Reduced graphene oxide (RGO) with nanocomposite polymer helped myocyte differentiation and skeletal muscle regeneration, angiogenesis, and functional recovery in vivo[147]. Carbon nanotubes have exceptionally strong electroconductive abilities and have potential to be used for implanted cell tracking and cellular behavior sensing, but they also possess potential cytotoxicity[148-150]. Conductive nanopolymers, such as PCL, have modifiable physical properties and can be used in composite hydrogels or electrospun nanofibers to enhance myoblast differentiation and functional maturation[151-155]. Murine myoblasts cultured in vitro on composite gelatin-polyaniline electrospun nanofibers demonstrated improved myotube contractility[155]. More recently, an elastic, hemostatic and conductive nanocomposite cryogel composed of RGO and gelatin exhibited significant cell proliferation, myogenic differentiation, and increased repair efficiency in a rat VML model[156]. An injectable electroconductive, biodegradable hydrogel with murine myoblasts showed higher myofiber density and capillary density in a rat TA VML model[157].
3D BIOPRINTING AND BIOINKS
In comparison to traditional tissue engineering strategies, 3D bioprinting using bioinks (combinations of scaffolds, cells, and growth factors) replicates the complex structure of skeletal muscle while precisely controlling the spatial positioning of cells and biomaterials [Figure 4][123,126,158]. Non-bioprinted biomaterial scaffolds fail to regain normal physiologic force generation and mature functional constructs and are limited in the ability to direct biomolecule deposition[126]. An in vivo nanocomposite VEGF-eluting hydrogel bioink demonstrated adherence to skeletal muscle and improved functional recovery with reduced fibrosis in a murine model of VML[159]. Other bioprinted scaffolds include a decellularized bioink that allowed for high cell viability, enhanced tissue and nerve vascularization, and functional recovery in a rat VML model and an in vivo colloidal foam-based porous hydrogel that showed significant functional restoration and force generation[160,161]. A methacrylated gelatin hydrogel with human adipose-derived cells, developed using an in situ crosslinking strategy to prevent loss of cell viability, showed improvements in hindlimb grip strength and muscular volume in a murine TA VML model[162]. One study involving a bioprinted acellular gelatin hydrogel with MPCs demonstrated TA muscle functional recovery of 82% in a rat TA VML model at eight weeks[163]. Functional neural integration of 3D bioprinted scaffolds has also recently been studied but still remains a challenge for engineered skeletal muscle[163]. A pre-innervated 3D bioprinted scaffold with human MPCs and human neural stem cells showed accelerated functional restoration by integration with host neurons and improved myofiber and NMJ formation in a rat model of VML[164]. Three-dimensional bioprinted scaffolds hold great promise, but scaffold immunocompatibility, systemic effects of implanted cells, and ability to bioprint thick skeletal muscle > 1 mm to allow for vascularization need to be investigated[126,163]. Bioprinting patient-derived stem cells and the development of various combinations of bioinked materials and cells require further direction and study[126].
Figure 4. Diagram of a handheld 3D printer used to print scaffolds directly into the muscle defect[165].
BARRIERS TO CLINICAL TRANSLATION
Current research on tissue-engineered skeletal muscle constructs for VML is mostly limited to small animal models [Table 3]. A few human clinical studies on decellularized ECM scaffolds derived from animal tissues have shown limited success in the restoration of muscle function[8,112]. Implantation of an acellular porcine-derived scaffold in a 13-patient cohort study showed an average improvement of 37.3% in strength, 27.1% improvement in functional range-of-motion tasks, and 27.2% increase in bulk muscle at six months[112]. Muscles injured included the anterior tibial compartment, brachialis, biceps, deltoid, quadriceps, rectus femoris, sartorius, and hamstring, with an average tissue deficit of 66.2% and ranged from 25%-90% compared to the contralateral muscle at enrollment into the study. By 24-28 weeks, strength testing ranged from -17.88% to 136.1% and improvements in force production in 8 of the 13 patients and overall significant improvement of 37.3% ± 12.4% and range-of-motion in at least one task improved by 27.1% ± 10.5% for all the patients[112]. By eight months, bulk muscle, identified by dense tissue on imaging, showed an average increase of 27.2%. Prior to ECM implantation, all the patients had personalized physical therapy regimens and standard-of-care treatments as well. However, the range of improvements varied widely among patients, and it is difficult to compare between patients with different injured muscles. The improvements in strength, range of motion, and bulk muscle, although positive, are still insufficient to fully restore muscle functionality to its pre-injury state.
Comparison of animal models of VML
Animal model | Advantages | Disadvantages |
Mouse | -Cost-effective and readily available[108] -Easily reproducible injury -Ability to obtain a large cohort | -Smaller scale defect than seen clinically[171] -Limited translational capacity to humans |
Rat | -Larger than mouse model -Easily reproducible injury -Physiologically, morphologically, and genetically more similar to humans compared to mice | -Smaller scale defect than seen clinically[171] -Limited translational capacity to humans |
Sheep /Pigs /Canine | -Larger size defect for more clinically relevant applications | -Limited by price, resources needed to care for them -Few large animal models |
Here, it is important to mention the incorporation of physical therapy to augment functional recovery following VML. Some studies in rodent models have demonstrated synergistic improvements in muscle strength by adding an exercise regimen[10,165,166]. One study involving a 3D bioprinted scaffold composed of gelatin methacryloyl with colloidal foam-like porosity incorporated progressive aerobic exercise using an 8-week treadmill running regimen and found a significant 25% improvement in tetanic gastrocnemius strength compared to the same treatment group without exercise in a murine gastrocnemius VML model[165]. Scaffold implantation, in combination with exercise training, synergistically improved functional recovery[165]. Similarly, one study involving a rat TA VML injury model alone, without the use of scaffolds, found a 17% improvement in maximal isometric torque after providing free-reign access to running wheels[166]. Another study evaluated early rehabilitation therapy of passive range of motion in a murine posterior compartment VML model and found 3-fold reduced muscle stiffness compared to VML alone[10].
In addition to the role of physical therapy, the use of electrical stimulation on muscle regeneration can further augment functional recovery. Intermittent electrical stimulation can potentially enhance the strength of the remaining muscle post-VML injury. The previous study involving early rehabilitation incorporated a regimen of passive range of motion with electrical stimulation and demonstrated 32% greater isometric plantarflexion torque compared to VML alone and 21% greater compared to range of motion therapy alone[10]. Clinically, early mobilization and therapy lead to improved function and recovery[166]. Further preclinical studies that incorporate exercise and physical therapy with scaffold implantation can hopefully translate to improved functional recovery in clinical patients. Challenges still remain for VML therapy to gain greater functional improvements and large volume muscle tissue.
The ideal scaffold with the optimum microarchitecture (porosity, elasticity, biodegradability, anisotropic), progenitor cell population, and combination of growth factors to effectively guide myogenesis in vivo is yet to be designed[131,167]. Vascularization, innervation, and immunocompatibility are essential for scaffold success, and no tissue engineering technology has been fully successful[60,64,167]. Force generation by engineered muscle tissue is reduced on strength testing compared to that of natural muscle[126]. Regeneration of large quantities of aligned myofibers for clinically sufficient functional restoration following scaffold implantation has yet to be achieved[126]. A better understanding of intricate spatiotemporal events in skeletal muscle regeneration and subsequent application to tissue-engineered scaffolds are needed[12,60]. Successful engineered scaffolds for tissue regeneration necessitate the formation of large volumes of autologous myoblasts, growth-factor delivery to support integration and survival of implanted cells in vivo, vessel and nerve vascularization, and immunomodulation to prevent excessive scar[22,120,139,168-170]. If scaffolds are cellular, rejection following scaffold implantation must also be considered. Scalability and accurate representation of tissue engineered constructs in VML animal models to human patients present another major challenge in clinical applications[170]. Muscle defects in mice and rats, the most used VML models, are much smaller than those seen clinically, and increasing scaffold size for clinical use will need effective strategies to promote angiogenesis, myogenesis, and neural integration within the construct. Variability in animal models, anatomic location and creation of muscle defects, and muscle function and recovery assessment tools can all lead to variable preclinical results, further limiting the translation from these studies to clinical settings[171]. Additionally, the pathway to industrialization and commercialization of tissue-engineered scaffolds requires improvements in efficient, quick, and cost-effective methods of manufacturing with thorough clinical trial testing that shows acceptable patient safety and clinical effectiveness from regulators and clinicians[158,172].
CONCLUSIONS AND FUTURE DIRECTIONS
While there is a current paucity of options for VML treatment, tissue engineering techniques offer opportunities to promote myogenesis and fibrosis following VML injury. The current standard of care using autologous functional muscle transfer is limited by the degree of functional recovery and donor site morbidity. Development of effective treatments to address large deficits in skeletal muscle mass is hopeful with tissue engineering. Bioengineered scaffolds can mimic native ECM and incorporate biophysical and biochemical cues to guide host cellular responses and functions, resulting in improved functional recovery. Translation into human patients has been achieved in thirteen patients so far with an acellular scaffold and physical therapy[111,112]. Clinical translation of scaffold treatment in patients with VML injuries could resemble the following paradigm: wound debridement, assessment of strength and range-of-motion, tissue-engineered scaffold implantation, and lastly, functional muscle flap coverage. With bioengineered scaffold implantation, patients with extremity VML injuries can achieve improved muscle functionality and, subsequently, a better quality of life. A combination of extensive physical therapy, scaffold implantation, and functional muscle transfer has the potential as a viable treatment option for VML. Although many challenges remain, further research in this area may allow for scaffolds to emerge as clinically useful treatment modalities for VML injury.
DECLARATIONS
Authors’ contributions
Made substantial contributions to writing the manuscript: Zhu C, Sklyar K, Karvar M
Provided revisions, guidance, and direction: Endo Y, Sinha I
Availability of data and materials
Not applicable.
Financial support and sponsorship
None.
Conflicts of interest
Indranil Sinha reports a relationship with InPrint Bio LLC that includes equity or stocks. The authors have no other relevant affiliations or financial involvement with any organization or entity with a financial interest in or financial conflict with the subject matter or materials discussed in the manuscript apart from those disclosed. All other authors declare that there are no conflicts of interest.
Ethical approval and consent to participate
All animal procedures were approved by the Institutional Animal Use and Care Committee of Harvard Medical School (Protocol number: 2016N000375).
Consent for publication
Not applicable.
Copyright
© The Author(s) 2023.
REFERENCES
1. Corona BT, Rivera JC, Greising SM. Inflammatory and physiological consequences of debridement of fibrous tissue after volumetric muscle loss injury. Clin Transl Sci 2018;11:208-17.
2. Corona BT, Rivera JC, Owens JG, Wenke JC, Rathbone CR. Volumetric muscle loss leads to permanent disability following extremity trauma. J Rehabil Res Dev 2015;52:785-92.
3. Corona BT, Wenke JC, Ward CL. Pathophysiology of volumetric muscle loss injury. Cells Tissues Organs 2016;202:180-8.
4. Garg K, Ward CL, Hurtgen BJ, et al. Volumetric muscle loss: persistent functional deficits beyond frank loss of tissue. J Orthop Res 2015;33:40-6.
5. Larouche J, Greising SM, Corona BT, Aguilar CA. Robust inflammatory and fibrotic signaling following volumetric muscle loss: a barrier to muscle regeneration. Cell Death Dis 2018;9:409.
6. Terada N, Takayama S, Yamada H, Seki T. Muscle repair after a transsection injury with development of a gap: an experimental study in rats. Scand J Plast Reconstr Surg Hand Surg 2001;35:233-8.
7. Anderson SE, Han WM, Srinivasa V, et al. Determination of a critical size threshold for volumetric muscle loss in the mouse quadriceps. Tissue Eng Part C Methods 2019;25:59-70.
8. Mase VJ Jr, Hsu JR, Wolf SE, et al. Clinical application of an acellular biologic scaffold for surgical repair of a large, traumatic quadriceps femoris muscle defect. Orthopedics 2010;33:511.
9. Cross JD, Ficke JR, Hsu JR, Masini BD, Wenke JC. Battlefield orthopaedic injuries cause the majority of long-term disabilities. J Am Acad Orthop Surg 2011;19 Suppl 1:S1-7.
10. Greising SM, Warren GL, Southern WM, et al. Early rehabilitation for volumetric muscle loss injury augments endogenous regenerative aspects of muscle strength and oxidative capacity. BMC Musculoskelet Disord 2018;19:173.
11. Porzionato A, Sfriso MM, Pontini A, et al. Decellularized human skeletal muscle as biologic scaffold for reconstructive surgery. Int J Mol Sci 2015;16:14808-31.
12. Bach AD, Beier JP, Stern-Staeter J, Horch RE. Skeletal muscle tissue engineering. J Cell Mol Med 2004;8:413-22.
13. Lin CH, Lin YT, Yeh JT, Chen CT. Free functioning muscle transfer for lower extremity posttraumatic composite structure and functional defect. Plast Reconstr Surg 2007;119:2118-26.
14. Ulusal AE, Lin CH, Lin YT, Ulusal BG, Yazar S. The use of free flaps in the management of type IIIB open calcaneal fractures. Plast Reconstr Surg 2008;121:2010-9.
15. Doi K, Hattori Y, Tan SH, Dhawan V. Basic science behind functioning free muscle transplantation. Clin Plast Surg 2002;29:483-95, v-vi.
16. Lee KT, Lee YJ, Kim A, Mun GH. Evaluation of donor morbidity following single-stage latissimus dorsi neuromuscular transfer for facial reanimation. Plast Reconstr Surg 2019;143:152e-64e.
17. Diwan A, Eberlin KR, Smith RM. The principles and practice of open fracture care, 2018. Chin J Traumatol 2018;21:187-92.
18. Mulbauer GD, Matthew HWT. Biomimetic scaffolds in skeletal muscle regeneration. Discoveries 2019;7:e90.
19. Panayi AC, Smit L, Hays N, et al. A porous collagen-GAG scaffold promotes muscle regeneration following volumetric muscle loss injury. Wound Repair Regen 2020;28:61-74.
20. Shayan M, Huang NF. Pre-clinical cell therapeutic approaches for repair of volumetric muscle loss. Bioengineering 2020;7:97.
21. Das S, Browne KD, Laimo FA, et al. Pre-innervated tissue-engineered muscle promotes a pro-regenerative microenvironment following volumetric muscle loss. Commun Biol 2020;3:330.
22. Aguilar CA, Greising SM, Watts A, et al. Multiscale analysis of a regenerative therapy for treatment of volumetric muscle loss injury. Cell Death Discov 2018;4:33.
23. Grogan BF, Hsu JR. Skeletal Trauma Research Consortium. Volumetric muscle loss. J Am Acad Orthop Surg 2011;19 Suppl 1:S35-7.
24. Forcina L, Miano C, Pelosi L, Musarò A. An overview about the biology of skeletal muscle satellite cells. Curr Genomics 2019;20:24-37.
25. Cezar CA, Mooney DJ. Biomaterial-based delivery for skeletal muscle repair. Adv Drug Deliv Rev 2015;84:188-97.
26. Tidball JG. Mechanisms of muscle injury, repair, and regeneration. Compr Physiol 2011;1:2029-62.
27. Grasman JM, Zayas MJ, Page RL, Pins GD. Biomimetic scaffolds for regeneration of volumetric muscle loss in skeletal muscle injuries. Acta Biomater 2015;25:2-15.
28. Nuutila K, Sakthivel D, Kruse C, Tran P, Giatsidis G, Sinha I. Gene expression profiling of skeletal muscle after volumetric muscle loss. Wound Repair Regen 2017;25:408-13.
29. Chargé SB, Rudnicki MA. Cellular and molecular regulation of muscle regeneration. Physiol Rev 2004;84:209-38.
30. Wozniak AC, Anderson JE. Nitric oxide-dependence of satellite stem cell activation and quiescence on normal skeletal muscle fibers. Dev Dyn 2007;236:240-50.
31. Allen RE, Sheehan SM, Taylor RG, Kendall TL, Rice GM. Hepatocyte growth factor activates quiescent skeletal muscle satellite cells in vitro. J Cell Physiol 1995;165:307-12.
32. Gal-Levi R, Leshem Y, Aoki S, Nakamura T, Halevy O. Hepatocyte growth factor plays a dual role in regulating skeletal muscle satellite cell proliferation and differentiation. Biochim Biophys Acta 1998;1402:39-51.
33. Tatsumi R, Anderson JE, Nevoret CJ, Halevy O, Allen RE. HGF/SF is present in normal adult skeletal muscle and is capable of activating satellite cells. Dev Biol 1998;194:114-28.
34. Tatsumi R, Hattori A, Ikeuchi Y, Anderson JE, Allen RE. Release of hepatocyte growth factor from mechanically stretched skeletal muscle satellite cells and role of pH and nitric oxide. Mol Biol Cell 2002;13:2909-18.
35. Järvinen TA, Järvinen TL, Kääriäinen M, Kalimo H, Järvinen M. Muscle injuries: biology and treatment. Am J Sports Med 2005;33:745-64.
36. Cornelison DD, Wilcox-Adelman SA, Goetinck PF, Rauvala H, Rapraeger AC, Olwin BB. Essential and separable roles for Syndecan-3 and Syndecan-4 in skeletal muscle development and regeneration. Genes Dev 2004;18:2231-6.
37. Cornelison DD, Filla MS, Stanley HM, Rapraeger AC, Olwin BB. Syndecan-3 and syndecan-4 specifically mark skeletal muscle satellite cells and are implicated in satellite cell maintenance and muscle regeneration. Dev Biol 2001;239:79-94.
38. Smith CW, Klaasmeyer JG, Woods TL, Jones SJ. Effects of IGF-I, IGF-II, bFGF and PDGF on the initiation of mRNA translation in C2C12 myoblasts and differentiating myoblasts. Tissue Cell 1999;31:403-12.
39. Dhawan J, Rando TA. Stem cells in postnatal myogenesis: molecular mechanisms of satellite cell quiescence, activation and replenishment. Trends Cell Biol 2005;15:666-73.
40. Grefte S, Kuijpers-Jagtman AM, Torensma R, Von den Hoff JW. Skeletal muscle development and regeneration. Stem Cells Dev 2007;16:857-68.
41. Folkman J, Klagsbrun M, Sasse J, Wadzinski M, Ingber D, Vlodavsky I. A heparin-binding angiogenic protein--basic fibroblast growth factor--is stored within basement membrane. Am J Pathol 1988;130:393-400.
42. DO MK, Suzuki T, Gerelt B, et al. Time-coordinated prevalence of extracellular HGF, FGF2 and TGF-β3 in crush-injured skeletal muscle. Anim Sci J 2012;83:712-7.
44. Laumonier T, Menetrey J. Muscle injuries and strategies for improving their repair. J Exp Orthop 2016;3:15.
45. Dhandayuthapani B, Yoshida Y, Maekawa T, Kumar DS. Polymeric scaffolds in tissue engineering application: a review. Int J Polym Sci 2011;2011:290602.
46. Webster MT, Manor U, Lippincott-Schwartz J, Fan CM. Intravital imaging reveals ghost fibers as architectural units guiding myogenic progenitors during regeneration. Cell Stem Cell 2016;18:243-52.
47. Qazi TH, Mooney DJ, Pumberger M, Geissler S, Duda GN. Biomaterials based strategies for skeletal muscle tissue engineering: existing technologies and future trends. Biomaterials 2015;53:502-21.
48. Mertens JP, Sugg KB, Lee JD, Larkin LM. Engineering muscle constructs for the creation of functional engineered musculoskeletal tissue. Regen Med 2014;9:89-100.
49. Sicari BM, Londono R, Badylak SF. Strategies for skeletal muscle tissue engineering: seed vs. soil. J Mater Chem B 2015;3:7881-95.
50. Zhuang P, An J, Chua CK, Tan LP. Bioprinting of 3D in vitro skeletal muscle models: a review. Materials & Design 2020;193:108794.
51. Bian W, Bursac N. Tissue engineering of functional skeletal muscle: challenges and recent advances. IEEE Eng Med Biol Mag 2008;27:109-13.
52. Baiguera S, Del Gaudio C, Di Nardo P, Manzari V, Carotenuto F, Teodori L. 3D printing decellularized extracellular matrix to design biomimetic scaffolds for skeletal muscle tissue engineering. Biomed Res Int 2020;2020:2689701.
53. Evans DJ, Britland S, Wigmore PM. Differential response of fetal and neonatal myoblasts to topographical guidance cues in vitro. Dev Genes Evol 1999;209:438-42.
54. Miyoshi H, Adachi T. Topography design concept of a tissue engineering scaffold for controlling cell function and fate through actin cytoskeletal modulation. Tissue Eng Part B Rev 2014;20:609-27.
55. Fan J, Abedi-Dorcheh K, Sadat Vaziri A, et al. A review of recent advances in natural polymer-based scaffolds for musculoskeletal tissue engineering. Polymers 2022;14:2097.
56. Jenkins TL, Little D. Synthetic scaffolds for musculoskeletal tissue engineering: cellular responses to fiber parameters. NPJ Regen Med 2019;4:15.
57. Cao H, Duan L, Zhang Y, Cao J, Zhang K. Current hydrogel advances in physicochemical and biological response-driven biomedical application diversity. Signal Transduct Target Ther 2021;6:426.
58. Loh QL, Choong C. Three-dimensional scaffolds for tissue engineering applications: role of porosity and pore size. Tissue Eng Part B Rev 2013;19:485-502.
59. Hutmacher DW. Scaffold design and fabrication technologies for engineering tissues--state of the art and future perspectives. J Biomater Sci Polym Ed 2001;12:107-24.
60. Corona BT, Greising SM. Challenges to acellular biological scaffold mediated skeletal muscle tissue regeneration. Biomaterials 2016;104:238-46.
61. Boldrin L, Elvassore N, Malerba A, et al. Satellite cells delivered by micro-patterned scaffolds: a new strategy for cell transplantation in muscle diseases. Tissue Eng 2007;13:253-62.
62. Zhu C, Karvar M, Koh DJ, et al. Acellular collagen-glycosaminoglycan matrix promotes functional recovery in a rat model of volumetric muscle loss. Regen Med 2023;18:623-33.
63. Badylak SF, Dziki JL, Sicari BM, Ambrosio F, Boninger ML. Mechanisms by which acellular biologic scaffolds promote functional skeletal muscle restoration. Biomaterials 2016;103:128-36.
64. Sicari BM, Dearth CL, Badylak SF. Tissue engineering and regenerative medicine approaches to enhance the functional response to skeletal muscle injury. Anat Rec 2014;297:51-64.
65. Menetrey J, Kasemkijwattana C, Day CS, et al. Growth factors improve muscle healing in vivo. J Bone Joint Surg Br 2000;82:131-7.
66. Ju YM, Atala A, Yoo JJ, Lee SJ. In situ regeneration of skeletal muscle tissue through host cell recruitment. Acta Biomater 2014;10:4332-9.
67. Silva EA, Mooney DJ. Spatiotemporal control of vascular endothelial growth factor delivery from injectable hydrogels enhances angiogenesis. J Thromb Haemost 2007;5:590-8.
68. Shvartsman D, Storrie-White H, Lee K, et al. Sustained delivery of VEGF maintains innervation and promotes reperfusion in ischemic skeletal muscles via NGF/GDNF signaling. Mol Ther 2014;22:1243-53.
69. Lee J, Bhang SH, Park H, Kim BS, Lee KY. Active blood vessel formation in the ischemic hindlimb mouse model using a microsphere/hydrogel combination system. Pharm Res 2010;27:767-74.
70. Frey SP, Jansen H, Raschke MJ, Meffert RH, Ochman S. VEGF improves skeletal muscle regeneration after acute trauma and reconstruction of the limb in a rabbit model. Clin Orthop Relat Res 2012;470:3607-14.
71. Hammers DW, Sarathy A, Pham CB, Drinnan CT, Farrar RP, Suggs LJ. Controlled release of IGF-I from a biodegradable matrix improves functional recovery of skeletal muscle from ischemia/reperfusion. Biotechnol Bioeng 2012;109:1051-9.
72. Doi K, Ikeda T, Marui A, et al. Enhanced angiogenesis by gelatin hydrogels incorporating basic fibroblast growth factor in rabbit model of hind limb ischemia. Heart Vessels 2007;22:104-8.
73. Layman H, Spiga MG, Brooks T, Pham S, Webster KA, Andreopoulos FM. The effect of the controlled release of basic fibroblast growth factor from ionic gelatin-based hydrogels on angiogenesis in a murine critical limb ischemic model. Biomaterials 2007;28:2646-54.
74. Yasuda Y, Koyama H, Tabata Y, et al. Controlled delivery of bFGF remodeled vascular network in muscle flap and increased perfusion capacity via minor pedicle. J Surg Res 2008;147:132-7.
75. Grasman JM, Do DM, Page RL, Pins GD. Rapid release of growth factors regenerates force output in volumetric muscle loss injuries. Biomaterials 2015;72:49-60.
76. Lee K, Silva EA, Mooney DJ. Growth factor delivery-based tissue engineering: general approaches and a review of recent developments. J R Soc Interface 2011;8:153-70.
77. Borselli C, Storrie H, Benesch-Lee F, et al. Functional muscle regeneration with combined delivery of angiogenesis and myogenesis factors. Proc Natl Acad Sci USA 2010;107:3287-92.
78. Skuk D, Goulet M, Roy B, et al. Dystrophin expression in muscles of duchenne muscular dystrophy patients after high-density injections of normal myogenic cells. J Neuropathol Exp Neurol 2006;65:371-86.
79. Saxena AK, Marler J, Benvenuto M, Willital GH, Vacanti JP. Skeletal muscle tissue engineering using isolated myoblasts on synthetic biodegradable polymers: preliminary studies. Tissue Eng 1999;5:525-32.
80. Baker HB, Passipieri JA, Siriwardane M, et al. Cell and growth factor-loaded keratin hydrogels for treatment of volumetric muscle loss in a mouse model. Tissue Eng Part A 2017;23:572-84.
81. Tomblyn S, Pettit Kneller EL, Walker SJ, et al. Keratin hydrogel carrier system for simultaneous delivery of exogenous growth factors and muscle progenitor cells. J Biomed Mater Res B Appl Biomater 2016;104:864-79.
82. Dellavalle A, Maroli G, Covarello D, et al. Pericytes resident in postnatal skeletal muscle differentiate into muscle fibres and generate satellite cells. Nat Commun 2011;2:499.
83. Crisan M, Yap S, Casteilla L, et al. A perivascular origin for mesenchymal stem cells in multiple human organs. Cell Stem Cell 2008;3:301-13.
84. Qu-Petersen Z, Deasy B, Jankowski R, et al. Identification of a novel population of muscle stem cells in mice: potential for muscle regeneration. J Cell Biol 2002;157:851-64.
85. Genovese P, Patel A, Ziemkiewicz N, et al. Co-delivery of fibrin-laminin hydrogel with mesenchymal stem cell spheroids supports skeletal muscle regeneration following trauma. J Tissue Eng Regen Med 2021;15:1131-43.
86. Chen FM, Zhang M, Wu ZF. Toward delivery of multiple growth factors in tissue engineering. Biomaterials 2010;31:6279-308.
87. Bao W, Li M, Yang Y, et al. Advancements and frontiers in the high performance of natural hydrogels for cartilage tissue engineering. Front Chem 2020;8:53.
88. Spicer CD. Hydrogel scaffolds for tissue engineering: the importance of polymer choice. Polym Chem 2020;11:184-219.
89. Chai Q, Jiao Y, Yu X. Hydrogels for biomedical applications: their characteristics and the mechanisms behind them. Gels 2017;3:6.
90. Nakayama KH, Shayan M, Huang NF. Engineering biomimetic materials for skeletal muscle repair and regeneration. Adv Healthc Mater 2019;8:e1801168.
91. Pollot BE, Rathbone CR, Wenke JC, Guda T. Natural polymeric hydrogel evaluation for skeletal muscle tissue engineering. J Biomed Mater Res B Appl Biomater 2018;106:672-9.
92. Billiet T, Vandenhaute M, Schelfhout J, Van Vlierberghe S, Dubruel P. A review of trends and limitations in hydrogel-rapid prototyping for tissue engineering. Biomaterials 2012;33:6020-41.
93. Carleton MM, Locke M, Sefton MV. Methacrylic acid-based hydrogels enhance skeletal muscle regeneration after volumetric muscle loss in mice. Biomaterials 2021;275:120909.
94. Passipieri JA, Baker HB, Siriwardane M, et al. Keratin hydrogel enhances in vivo skeletal muscle function in a rat model of volumetric muscle loss. Tissue Eng Part A 2017;23:556-71.
95. Juhas M, Engelmayr GC Jr, Fontanella AN, Palmer GM, Bursac N. Biomimetic engineered muscle with capacity for vascular integration and functional maturation in vivo. Proc Natl Acad Sci USA 2014;111:5508-13.
96. Beier JP, Stern-Straeter J, Foerster VT, Kneser U, Stark GB, Bach AD. Tissue engineering of injectable muscle: three-dimensional myoblast-fibrin injection in the syngeneic rat animal model. Plast Reconstr Surg 2006;118:1113-21.
97. Borselli C, Cezar CA, Shvartsman D, Vandenburgh HH, Mooney DJ. The role of multifunctional delivery scaffold in the ability of cultured myoblasts to promote muscle regeneration. Biomaterials 2011;32:8905-14.
98. Wang L, Cao L, Shansky J, Wang Z, Mooney D, Vandenburgh H. Minimally invasive approach to the repair of injured skeletal muscle with a shape-memory scaffold. Mol Ther 2014;22:1441-9.
99. Rossi CA, Flaibani M, Blaauw B, et al. In vivo tissue engineering of functional skeletal muscle by freshly isolated satellite cells embedded in a photopolymerizable hydrogel. FASEB J 2011;25:2296-304.
100. Wang HD, Lough DM, Kurlander DE, Lopez J, Quan A, Kumar AR. Muscle-derived stem cell-enriched scaffolds are capable of enhanced healing of a murine volumetric muscle loss defect. Plast Reconstr Surg 2019;143:329e-39e.
101. Malafaya PB, Silva GA, Reis RL. Natural-origin polymers as carriers and scaffolds for biomolecules and cell delivery in tissue engineering applications. Adv Drug Deliv Rev 2007;59:207-33.
102. Zhang X, Zhang R, Wu S, Sun Y, Yang H, Lin B. Physically and chemically dual-crosslinked hydrogels with superior mechanical properties and self-healing behavior. New J Chem 2020;44:9903-11.
103. Kim S, Regitsky AU, Song J, Ilavsky J, McKinley GH, Holten-Andersen N. In situ mechanical reinforcement of polymer hydrogels via metal-coordinated crosslink mineralization. Nat Commun 2021;12:667.
104. Hogrebe NJ, Reinhardt JW, Gooch KJ. Biomaterial microarchitecture: a potent regulator of individual cell behavior and multicellular organization. J Biomed Mater Res A 2017;105:640-61.
105. Xu J, Nie N, Wu B, et al. The personalized application of biomaterials based on age and sexuality specific immune responses. Biomaterials 2021;278:121177.
106. Jiang Z, Fu M, Zhu D, et al. Genetically modified immunomodulatory cell-based biomaterials in tissue regeneration and engineering. Cytokine Growth Factor Rev 2022;66:53-73.
107. Wu J, Matthias N, Bhalla S, Darabi R. Evaluation of the therapeutic potential of human iPSCs in a murine model of VML. Mol Ther 2021;29:121-31.
108. Valentin JE, Badylak JS, McCabe GP, Badylak SF. Extracellular matrix bioscaffolds for orthopaedic applications. a comparative histologic study. J Bone Joint Surg Am 2006;88:2673-86.
109. Sicari BM, Agrawal V, Siu BF, et al. A murine model of volumetric muscle loss and a regenerative medicine approach for tissue replacement. Tissue Eng Part A 2012;18:1941-8.
110. Porzionato A, Sfriso MM, Pontini A, et al. Decellularized human skeletal muscle as biologic scaffold for reconstructive surgery. Int J Mol Sci 2015;16:14808-31.
111. Aurora A, Roe JL, Corona BT, Walters TJ. An acellular biologic scaffold does not regenerate appreciable de novo muscle tissue in rat models of volumetric muscle loss injury. Biomaterials 2015;67:393-407.
112. Sicari BM, Rubin JP, Dearth CL, et al. An acellular biologic scaffold promotes skeletal muscle formation in mice and humans with volumetric muscle loss. Sci Transl Med 2014;6:234ra58.
113. Dziki J, Badylak S, Yabroudi M, et al. An acellular biologic scaffold treatment for volumetric muscle loss: results of a 13-patient cohort study. NPJ Regen Med 2016;1:16008.
114. Garg K, Ward CL, Rathbone CR, Corona BT. Transplantation of devitalized muscle scaffolds is insufficient for appreciable de novo muscle fiber regeneration after volumetric muscle loss injury. Cell Tissue Res 2014;358:857-73.
115. Kasukonis B, Kim J, Brown L, et al. Codelivery of infusion decellularized skeletal muscle with minced muscle autografts improved recovery from volumetric muscle loss injury in a rat model. Tissue Eng Part A 2016;22:1151-63.
116. Merritt EK, Cannon MV, Hammers DW, et al. Repair of traumatic skeletal muscle injury with bone-marrow-derived mesenchymal stem cells seeded on extracellular matrix. Tissue Eng Part A 2010;16:2871-81.
117. Conconi MT, De Coppi P, Bellini S, et al. Homologous muscle acellular matrix seeded with autologous myoblasts as a tissue-engineering approach to abdominal wall-defect repair. Biomaterials 2005;26:2567-74.
118. Krampera M, Pizzolo G, Aprili G, Franchini M. Mesenchymal stem cells for bone, cartilage, tendon and skeletal muscle repair. Bone 2006;39:678-83.
119. Tae SK, Lee SH, Park JS, Im GI. Mesenchymal stem cells for tissue engineering and regenerative medicine. Biomed Mater 2006;1:63-71.
120. De Coppi P, Bellini S, Conconi MT, et al. Myoblast-acellular skeletal muscle matrix constructs guarantee a long-term repair of experimental full-thickness abdominal wall defects. Tissue Eng 2006;12:1929-36.
121. Gilbert-Honick J, Grayson W. Vascularized and innervated skeletal muscle tissue engineering. Adv Healthc Mater 2020;9:e1900626.
122. Wang S, Yan H, Fang B, et al. A myogenic niche with a proper mechanical stress environment improves abdominal wall muscle repair by modulating immunity and preventing fibrosis. Biomaterials 2022;285:121519.
123. Ziemkiewicz N, Hilliard GM, Dunn AJ, et al. Laminin-111-enriched fibrin hydrogels enhance functional muscle regeneration following trauma. Tissue Eng Part A 2022;28:297-311.
124. Samandari M, Quint J, Rodríguez-delaRosa A, Sinha I, Pourquié O, Tamayol A. Bioinks and bioprinting strategies for skeletal muscle tissue engineering. Adv Mater 2022;34:e2105883.
125. Vasita R, Katti DS. Nanofibers and their applications in tissue engineering. Int J Nanomedicine 2006;1:15-30.
126. Kishan AP, Cosgriff-Hernandez EM. Recent advancements in electrospinning design for tissue engineering applications: a review. J Biomed Mater Res A 2017;105:2892-905.
127. Ostrovidov S, Salehi S, Costantini M, et al. 3D bioprinting in skeletal muscle tissue engineering. Small 2019;15:e1805530.
128. Jana S, Levengood SK, Zhang M. Anisotropic materials for skeletal-muscle-tissue engineering. Adv Mater 2016;28:10588-612.
129. Li WJ, Laurencin CT, Caterson EJ, Tuan RS, Ko FK. Electrospun nanofibrous structure: a novel scaffold for tissue engineering. J Biomed Mater Res 2002;60:613-21.
130. Wang L, Li T, Wang Z, et al. Injectable remote magnetic nanofiber/hydrogel multiscale scaffold for functional anisotropic skeletal muscle regeneration. Biomaterials 2022;285:121537.
131. Nakayama KH, Quarta M, Paine P, et al. Treatment of volumetric muscle loss in mice using nanofibrillar scaffolds enhances vascular organization and integration. Commun Biol 2019;2:170.
132. Wang L, Wu Y, Guo B, Ma PX. Nanofiber yarn/hydrogel core-shell scaffolds mimicking native skeletal muscle tissue for guiding 3D myoblast alignment, elongation, and differentiation. ACS Nano 2015;9:9167-79.
133. Lee JB, Jeong SI, Bae MS, et al. Highly porous electrospun nanofibers enhanced by ultrasonication for improved cellular infiltration. Tissue Eng Part A 2011;17:2695-702.
134. Leong MF, Rasheed MZ, Lim TC, Chian KS. In vitro cell infiltration and in vivo cell infiltration and vascularization in a fibrous, highly porous poly(D,L-lactide) scaffold fabricated by cryogenic electrospinning technique. J Biomed Mater Res A 2009;91:231-40.
135. Wright L, Andric T, Freeman J. Utilizing NaCl to increase the porosity of electrospun materials. Materials Science and Engineering: C 2011;31:30-6.
136. Soliman S, Pagliari S, Rinaldi A, et al. Multiscale three-dimensional scaffolds for soft tissue engineering via multimodal electrospinning. Acta Biomater 2010;6:1227-37.
137. Rnjak-Kovacina J, Weiss AS. Increasing the pore size of electrospun scaffolds. Tissue Eng Part B Rev 2011;17:365-72.
138. Jin G, Li K. The electrically conductive scaffold as the skeleton of stem cell niche in regenerative medicine. Mater Sci Eng C Mater Biol Appl 2014;45:671-81.
139. Saberi A, Jabbari F, Zarrintaj P, Saeb MR, Mozafari M. Electrically conductive materials: opportunities and challenges in tissue engineering. Biomolecules 2019;9:448.
140. Greising SM, Corona BT, McGann C, Frankum JK, Warren GL. Therapeutic approaches for volumetric muscle loss injury: a systematic review and meta-analysis. Tissue Eng Part B Rev 2019;25:510-25.
141. Farr AC, Hogan KJ, Mikos AG. Nanomaterial additives for fabrication of stimuli-responsive skeletal muscle tissue engineering constructs. Adv Healthc Mater 2020;9:e2000730.
142. Ito A, Yamamoto Y, Sato M, et al. Induction of functional tissue-engineered skeletal muscle constructs by defined electrical stimulation. Sci Rep 2014;4:4781.
143. Khodabukus A, Madden L, Prabhu NK, et al. Electrical stimulation increases hypertrophy and metabolic flux in tissue-engineered human skeletal muscle. Biomaterials 2019;198:259-69.
144. Kaji H, Ishibashi T, Nagamine K, Kanzaki M, Nishizawa M. Electrically induced contraction of C2C12 myotubes cultured on a porous membrane-based substrate with muscle tissue-like stiffness. Biomaterials 2010;31:6981-6.
145. Jo H, Sim M, Kim S, et al. Electrically conductive graphene/polyacrylamide hydrogels produced by mild chemical reduction for enhanced myoblast growth and differentiation. Acta Biomater 2017;48:100-9.
146. Shin SR, Aghaei-Ghareh-Bolagh B, Dang TT, et al. Cell-laden microengineered and mechanically tunable hybrid hydrogels of gelatin and graphene oxide. Adv Mater 2013;25:6385-91.
147. Chen C, Xi Y, Weng Y. Progress in the development of graphene-based biomaterials for tissue engineering and regeneration. Materials 2022;15:2164.
148. Du Y, Ge J, Li Y, Ma PX, Lei B. Biomimetic elastomeric, conductive and biodegradable polycitrate-based nanocomposites for guiding myogenic differentiation and skeletal muscle regeneration. Biomaterials 2018;157:40-50.
149. Harrison BS, Atala A. Carbon nanotube applications for tissue engineering. Biomaterials 2007;28:344-53.
150. Edwards SL, Werkmeister JA, Ramshaw JA. Carbon nanotubes in scaffolds for tissue engineering. Expert Rev Med Devices 2009;6:499-505.
151. Kobayashi N, Izumi H, Morimoto Y. Review of toxicity studies of carbon nanotubes. J Occup Health 2017;59:394-407.
152. Jun I, Jeong S, Shin H. The stimulation of myoblast differentiation by electrically conductive sub-micron fibers. Biomaterials 2009;30:2038-47.
153. Ku SH, Lee SH, Park CB. Synergic effects of nanofiber alignment and electroactivity on myoblast differentiation. Biomaterials 2012;33:6098-104.
154. Balint R, Cassidy NJ, Cartmell SH. Conductive polymers: towards a smart biomaterial for tissue engineering. Acta Biomater 2014;10:2341-53.
155. Chen MC, Sun YC, Chen YH. Electrically conductive nanofibers with highly oriented structures and their potential application in skeletal muscle tissue engineering. Acta Biomater 2013;9:5562-72.
156. Ostrovidov S, Ebrahimi M, Bae H, et al. Gelatin-polyaniline composite nanofibers enhanced excitation-contraction coupling system maturation in myotubes. ACS Appl Mater Interfaces 2017;9:42444-58.
157. Zhao X, Zhang Z, Luo J, et al. Biomimetic, highly elastic conductive and hemostatic gelatin/rGO-based nanocomposite cryogel to improve 3D myogenic differentiation and guide in vivo skeletal muscle regeneration. Applied Materials Today 2022;26:101365.
158. Guo B, Qu J, Zhao X, Zhang M. Degradable conductive self-healing hydrogels based on dextran-graft-tetraaniline and N-carboxyethyl chitosan as injectable carriers for myoblast cell therapy and muscle regeneration. Acta Biomater 2019;84:180-93.
159. Jessop ZM, Al-Sabah A, Gardiner MD, Combellack E, Hawkins K, Whitaker IS. 3D bioprinting for reconstructive surgery: principles, applications and challenges. J Plast Reconstr Aesthet Surg 2017;70:1155-70.
160. Quint JP, Mostafavi A, Endo Y, et al. In vivo printing of nanoenabled scaffolds for the treatment of skeletal muscle injuries. Adv Healthc Mater 2021;10:e2002152.
161. Choi YJ, Jun YJ, Kim DY, et al. A 3D cell printed muscle construct with tissue-derived bioink for the treatment of volumetric muscle loss. Biomaterials 2019;206:160-9.
162. Mostafavi A, Samandari M, Karvar M, et al. Colloidal multiscale porous adhesive (bio)inks facilitate scaffold integration. Appl Phys Rev 2021;8:041415.
163. Hwangbo H, Lee H, Jin EJ, et al. Bio-printing of aligned GelMa-based cell-laden structure for muscle tissue regeneration. Bioact Mater 2022;8:57-70.
164. Kim JH, Seol YJ, Ko IK, et al. 3D Bioprinted Human Skeletal Muscle Constructs for Muscle Function Restoration. Sci Rep 2018;8:12307.
165. Kim JH, Kim I, Seol YJ, et al. Neural cell integration into 3D bioprinted skeletal muscle constructs accelerates restoration of muscle function. Nat Commun 2020;11:1025.
166. Endo Y, Samandari M, Karvar M, et al. Aerobic exercise and scaffolds with hierarchical porosity synergistically promote functional recovery post volumetric muscle loss. Biomaterials 2023;296:122058.
167. Aurora A, Garg K, Corona BT, Walters TJ. Physical rehabilitation improves muscle function following volumetric muscle loss injury. BMC Sports Sci Med Rehabil 2014;6:41.
168. Edri R, Gal I, Noor N, et al. Personalized hydrogels for engineering diverse fully autologous tissue implants. Adv Mater 2019;31:e1803895.
169. Eugenis I, Wu D, Rando TA. Cells, scaffolds, and bioactive factors: Engineering strategies for improving regeneration following volumetric muscle loss. Biomaterials 2021;278:121173.
170. Greising SM, Rivera JC, Goldman SM, Watts A, Aguilar CA, Corona BT. Unwavering pathobiology of volumetric muscle loss injury. Sci Rep 2017;7:13179.
171. Bursac N, Juhas M, Rando TA. Synergizing engineering and biology to treat and model skeletal muscle injury and disease. Annu Rev Biomed Eng 2015;17:217-42.
172. Sicherer ST, Venkatarama RS, Grasman JM. Recent trends in injury models to study skeletal muscle regeneration and repair. Bioengineering 2020;7:76.
Cite This Article
Export citation file: BibTeX | RIS
OAE Style
Zhu C, Sklyar K, Karvar M, Endo Y, Sinha I. Scaffold tissue engineering strategies for volumetric muscle loss. Plast Aesthet Res 2023;10:58. http://dx.doi.org/10.20517/2347-9264.2022.89
AMA Style
Zhu C, Sklyar K, Karvar M, Endo Y, Sinha I. Scaffold tissue engineering strategies for volumetric muscle loss. Plastic and Aesthetic Research. 2023; 10: 58. http://dx.doi.org/10.20517/2347-9264.2022.89
Chicago/Turabian Style
Zhu, Christina, Karina Sklyar, Mehran Karvar, Yori Endo, Indranil Sinha. 2023. "Scaffold tissue engineering strategies for volumetric muscle loss" Plastic and Aesthetic Research. 10: 58. http://dx.doi.org/10.20517/2347-9264.2022.89
ACS Style
Zhu, C.; Sklyar K.; Karvar M.; Endo Y.; Sinha I. Scaffold tissue engineering strategies for volumetric muscle loss. Plast. Aesthet. Res. 2023, 10, 58. http://dx.doi.org/10.20517/2347-9264.2022.89
About This Article
Copyright
Data & Comments
Data
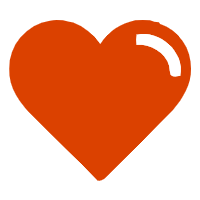

Comments
Comments must be written in English. Spam, offensive content, impersonation, and private information will not be permitted. If any comment is reported and identified as inappropriate content by OAE staff, the comment will be removed without notice. If you have any queries or need any help, please contact us at support@oaepublish.com.